Review
Rev Diabet Stud,
2019,
15:35-48 |
DOI 10.1900/RDS.2019.15.35 |
Impact of Physical Exercise on Gut Microbiome, Inflammation, and the Pathobiology of Metabolic Disorders
Muhammad U. Sohail1, Hadi M. Yassine1, Aaqib Sohail2, Asmaa A. Al Thani1
1Biomedical Research Center, Qatar University, P.O. Box 2713, Doha, Qatar
2Research Group Biomarkers for Infectious Diseases, TWINCORE Centre for Clinical and Experimental Infection Research, Hannover, Germany
Address correspondence to: Muhammad U. Sohail, Biomedical Research Center, Zone 5, H10 Building, Qatar University, PO Box 2713, Doha, Qatar, e-mail: drumarsohail@gmail.com
Manuscript submitted February 3, 2019; resubmitted May 15, 2019; accepted June 10, 2019.
Keywords: diabetes, exercise, microbiome, microbe, inflammation, immune response, obesity, oxidative stress
Abstract
BACKGROUND: The gastrointestinal tract (GIT) harbors a complex and diverse microbial composition that outnumbers our own body cells and their gene contents. These microbes play a significant role in host metabolism and energy homeostasis. Emerging evidence suggests that the GIT microbiome significantly contributes to host health and that impairments in the microbiome may cause the development of metabolic diseases. The microbiome architecture is shaped by several genetic and environmental factors, including nutrition and physical activity. Physical exercise has preventive or therapeutic effects in respiratory, cardiovascular, neuroendocrine, and muscular diseases. Yet, we still have little information of the beneficial effects of physical exercise on GIT health and microbial composition. Furthermore, we are not aware whether exercise-derived benefits on microbiome diversity can beneficially influence other tissues and body organs. OBJECTIVES: The aim of this article is to review the available literature on exercise-induced microbiome changes and to explain how these changes may induce inflammatory, immune, and oxidative responses that may contribute to the improvement of metabolic disorders. METHODS: A systemic and comprehensive search of the relevant literature using MEDLINE and Google Scholar databases was conducted during fall 2018 and spring 2019. The search identified sixty-two research and review articles that discussed exercise-induced microbiome changes. RESULTS: The review of the relevant literature suggests that exercise-induced microbial changes affect the host's immune pathways and improve energy homeostasis. Microbes release certain neuroendocrine and immune-modulatory factors that may lower inflammatory and oxidative stress and relieve patients suffering from metabolic disorders. CONCLUSIONS: Exercise-induced changes in microbial diversity are able to improve tissue metabolism, cardiorespiratory fitness, and insulin resistance.
Abbreviations: AMPK - 5′ AMP-activated protein kinase; BRFSS - Behavioral Risk Factor Surveillance System; BWG - body weight gain; CFU - coliform unit; GABA - γ-aminobutyric acid; GIT - gastrointestinal tract; GLP-1 - glucagon-like peptide-1; GPR - G protein receptor; GPR - G-protein-coupled receptor; HFD - high-fat diet; HPA - hypothalamus-pituitary-adrenal; IL - interleukin; LDL - low-density lipoprotein; LPS - lipopolysaccharides; NCD - non-communicable disease; NF-κB - nuclear factor kappa light-chain enhancer of activated B cells; ORMD - obesity-related metabolic disorder; PYY - peptide YY; SCFA - short-chain fatty acid; SGLT1 - sodium-glucose cotransporter 1; Th cell - T helper cell; TLR - toll-like receptor; Treg cell - T regulatory cell
1. Introduction
During the last century the mortality index indicated a gradual shift from infectious diseases towards non-communicable diseases (NCDs) [1]. Simultaneously, the importance of healthy lifestyle has been emphasized. Besides genetics, diet and lifestyle are the two most important factors associated with the risk of obesity-related metabolic disorders (ORMDs). Consumption of a calorie-rich diet and sedentary lifestyle have contributed to the rising incidence of ORMDs in industrialized countries. In particular, cardiovascular diseases, diabetes, and obesity have been associated with sedentary lifestyle.
Research on the gastrointestinal tract (GIT) microbiome has increased our understanding of the bi-directional communication between host and microbiome which is carried out through neuroendocrine signals and genetic transfer, and increased knowledge on "microbiome perturbations" in the pathogenesis of ORMDs [2]. Cumulative evidence suggests that the GIT microbiome is an essential mediator between different factors such as genetics, diet, exercise, and environment and the pathophysiology of the ORMDs [3]. Genetics, dietary habits, and host environment may influence the microbiome and eventually affect host metabolism. While this interaction has been studied extensively in diet-induced microbiome perturbations and host physiological and pathological processes [4], little attention has been given to other factors such as physical exercise.
GIT microbiome research has emerged as a potential exploratory field with special focus on ORMD pathogenesis. Microbiome dysbiosis (derangements in microbial ecology) in ORMDs has been reported [5]. Because of the presence of highly diverse microbial populations, the GIT represents the primary site of immunological activity. Lifestyle and physical activity may act as important factors to reshape gut microbiome and immunological responses [6], and provide the opportunity to influence disease development by therapeutic measures.
Therefore, in this article, we collected information on the impact of exercise on GIT microbiome and discussed how this information may relate to major NCDs, such as obesity and diabetes. We have previously reported changes in microbiome diversity in a type 1 diabetes rat model [7], and we have outlined the changes in microbiome architecture in diabetes and obesity [8]. In this review, we also analyzed exercise-associated changes in the microbiome that are able to modify body physiology and homeostasis.
2. Methods
The review of the literature was intended to identify taxonomic and community changes in the GIT microbiome in response to physical exercise. We explored exercise-mediated alterations in gut microbiome and metabolites that could be related to systemic inflammation, oxidative stress, and impaired immune responses. A systematic and comprehensive search of MEDLINE and Google Scholar was conducted between fall 2018 and spring 2019 to find relevant research articles published from 2000 to 2019. The following keywords were used in different combinations to retrieve all relevant research articles published in the English language:
- Exercise
- Physical activity
- Microbiome
- Metabolic disorders
- Inflammation
- Immunity
- Obesity
- Diabetes
References and bibliographic lists in published and review articles were also reviewed to identify relevant studies that may have been overlooked during database searches. All original research articles that presented data on intestinal microbiome, exercise, and/or metabolic disorders in humans and animals were included in the study. The literature search was not restricted to:
- Pattern, intensity, or duration of exercise
- Participants' gender or age
- Sampling pattern or
- Study design
We retrieved 51 original articles and 10 review papers published during 2008-2019 (Figure 1). The research articles were divided into the following two categories:
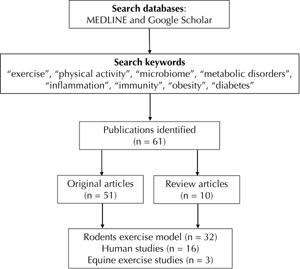 |
 |
Figure 1. The figure illustrates the search method used to collect research articles from Medline and Google Scholar databases. |
|
- Articles that studied exercise-induced microbiome diversity.
- Generic articles that linked exercise-induced microbiome changes with ORMDs.
For each study, we retrieved data on exercise pattern, microbiome diversity, metabolic responses, and immune-inflammatory markers. The primary outcome of this effort was in the form of a data base on the following aspects:
- GIT microbiome taxonomy and metabolic profile
- Immune-inflammatory responses
- Oxidative stress
A few studies discussed the molecular mechanisms involved in exercise-mediated alterations of the microbiome and systemic inflammation and immunity [9, 10], while the majority of studies simply described phylogenetic changes observed in response to exercise. To pursue the objective of this review, namely to find out more about the relation between microbiome changes and exercise with respect to metabolic diseases, we further explored review articles and mechanistic studies that related systemic inflammation and immunity to microbiome and exercise.
3. The microbiome as a virtual body organ
Most body surfaces of mammalians harbor a robust microbial colonization that is established to protect the body against potential pathogens; it is collectively called the microbiome. The microbiome is composed of archaea, bacteria, eukaryotes, viruses, and their genetic material. Both cell number and genetic material of the microbiome outnumber our own body cells and their genetic materials [11]. The host's genetics and several habitual and environmental factors act together in shaping host microbiome (Figure 2). Beyond genetics, a number of habitual and environmental factors contribute to shaping the host microbiome, including mode of delivery at birth, breastfeeding as opposed to formula feeding, adult dietary habits, hygiene, physical activity, antibiotics or xenobiotics exposure, and geographical location [12-14].
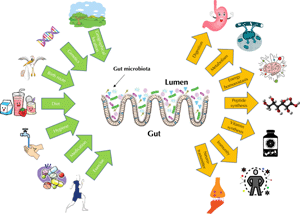 |
 |
Figure 2. Factors that influence the human microbiome architecture and different functions performed by our microbiome. Genetics and mode of delivery at birth are the first predisposing factors impacting the gastrointestinal (GIT) microbiome. They work together with breast-feeding, hygiene, exposure to medication (antibiotics), dietary habits, and physical exercise to shape GIT microbiome. The diagram also sketches the different functions of the GIT microbiome. The microbiome helps to digest food and control host body energy homeostasis. Microbes secrete several useful peptides and are part of our body’s largest immune system. |
|
The microbiome has essential structural, protective, metabolic, and endocrine functions that include food digestion, pathogen displacement, and nutrient synthesis [15, 16]. The GIT is the primary site of interaction between our body's largest component of the immune system and microbial colonization. The commensal microbes not only tune and train this local immunity, they also impact systemic immunity. Microbes interact with the nervous and endocrine system through glucagon-like peptide 1 (GLP-1) and peptide YY (PYY) that are produced in enteroendocrine L-cells, even though the exact mechanisms involved in this communication are not fully understood [17].
The endocrine activities of the microbiome are very heterogeneous. Frequently, the metabolic products of the microbiome, such as short-chain fatty acids (SCFAs), histamine, monoamines, and γ-aminobutyric acid (GABA), act as second messenger molecules or neurotransmitters [18-20]. These molecules mediate gut-brain and endocrine communications and contribute to the microbiome’s systemic functions. Gnotobiotic rodent models and probiotic supplementation studies have highlighted the significance of the microbiome. The poor microbial composition in gnotobiotic rats is explicated by averted hypothalamic-pituitary-adrenal (HPA) responses to psychological or environmental stressors [21, 22]. This neuroendocrine containment within the microbiome influences behavior and learning aptitude through biosynthesis of microbial neurochemicals either directly or by bi-directional transfer of genetic information between host and microbiome [23].
SCFAs are the main metabolic products of microbiome fermentation. These molecules act as signaling ligands for G-protein-coupled receptors (GPRs) on enterocytes for chemosensation, appetite regulation, and peripheral nutrient disposal [24]. Acetate, butyrate, and propionate are the main SCFAs that are produced by bacterial fermentation. Acetate and propionate enhance the expression of GPR-43 in immune cells to regulate immune-inflammatory responses [25]. Furthermore, these metabolites improve intestinal barrier function and display potent immunoregulatory properties through T cell proliferation and cytokine production [26].
4. Microbial dysbiosis and aberrant energy homeostasis: the prodrome of metabolic syndromes
Obesity is the consequence of genetic predisposition, chronic energy imbalance, and lack of proper physical activity. Extensive diet restriction as well as lifestyle, surgical, and pharmacological interventions have been implemented to treat obesity. These efforts are targeted at losing weight, but they have demonstrated insufficient, unsafe, and inconsistent results in most of the cases [27-29]. In recent years, significant progress has been made in the understanding of the GIT microbiome, suggesting a crucial intermediate role in energy homeostasis.
The basic functions of the gut microbiome are to digest dietary fibers and provide energy to the host. Changes in GIT microbiome composition alter digestive efficiency and consequently host metabolism [30]. Several studies have demonstrated substantial changes in microbiome composition in obese subjects compared with healthy controls, and these changes were associated with ORMDs. Palleja et al. observed that weight loss interventions are associated with beneficial changes in bacterial diversity and improvements in metabolic functions [31]. Furthermore, fecal microbiome transplant from lean donors recapitulates the functional metabolic phenotype and improves insulin sensitivity in the obese recipients [32].
onsumption of energy-rich food or antibiotics can induce microbiome perturbations and promote the incidence of metabolic syndromes. For example, prolonged antibiotic treatment, or antibiotic consumption during early life may induce microbial dysbiosis and promote obesity and diabetes [33, 34]. Hicks et al. observed a positive correlation between microbial dysbiosis, antibiotic consumption, and metabolic syndrome [35]. The authors performed a retrospective analysis using major data pools, including USA population data, American Community Survey, Area Resource File data, and the Behavioral Risk Factor Surveillance System (BRFSS) data obtained from Centers for Disease Control and Prevention. They found that the obese portion of the American population has the highest antibiotic prescription rate.
While these studies suggest an association between microbiome and host energy homeostasis, most of them lack a mechanistic explanation of the impact of microbiome changes on host energy cycle. It is still unknown whether these microbiome changes are cause or effect of obesity. We present the most discussed microbiome-energy homeostasis pathways below.
Recent evidence suggests bi-directional communication, through neuroendocrine signals, between gut microbiota and host cell energy homeostasis [36]. Several high-impact studies link SCFA production in gut fermentation to host energy homeostasis [37, 38]. Furthermore, microbiome composition is a key regulator of non-digestible carbohydrate and fiber digestion capacity. Cani et al. have discussed the microbiome's capacity to digest fibers, the production of SCFAs, and host energy homeostasis in symbiotic relationship [39]. In brief, SCFAs promote mitochondrial function, lipolysis, neoglucogenesis, beige adipogenesis, and release of GLP-1 and PYY. Conversely, these intestinal hormones influence food intake by modulating the activity of brain feeding centers. Furthermore, as revealed by germ-free mice experiments, microbial fermentation products stimulate the production of leptin in adipocytes that promote hepatic lipogenesis and surplus energy storage [40].
5. Exercise as a modulator of gut microbiome
There is compelling evidence to suggest that long-term exercise has a positive effect on energy homeostasis and plays a preventive role against various ORMDs. The most important physiological adaptations that occur in response to exercise are mitochondrial biogenesis, muscular hypertrophy and angiogenesis, cardiovascular fitness, better organized energy supply chain, and activation of the HPA axis. Exercise-induced activation of the HPA axis improves energy balance and better regulates immune-inflammatory responses [20]. Exercise promotes the cholinergic anti-inflammatory pathway and reduces tumor necrosis factor α (TNF-α) release. Lowder et al. reported that continuous moderate exercise is able to prevent influenza infection in rats by enhancing the production of anti-inflammatory cytokines (IL-10 and IL-4) and by lowering the secretion of pro-inflammatory cytokines (TNF-α, IL-1, interferon-γ) [41].
Since 2008, more than 50 research articles have been published on the impact of exercise on gut microbiome diversity. Effects of exercise on GIT health, though not fully explained, have emerged as a key interest in cancer and metabolic disease research. Endurance exercise may modulate GIT immune-inflammatory and redox responses, GIT permeability, motility, and stool transit time and consistency [42-44]. Hoffmann et al. reported that acute and continuing exercise regimes of varying intensities could increase antioxidant capacity, lymphocyte turnover, and expression of anti-inflammatory cytokine in the intestinal wall [43]. In the initial stages of intense exercise, GIT hypoxia and hypoperfusion may increase gut permeability, endotoxemia and oxidative stress [45, 46]. However, these changes in inflammation and immune response are transient and subside quickly. In particular, these changes are associated with the GIT microbiome in one way or another. It is also observed that specifically designed exercise therapies mitigate GIT inflammatory diseases by preferentially modulating microbiome diversity and metabolic profile [47, 48]. It is suggested that exercise increases gut motility, which may increase the shedding of loosely bound microbes in the GIT epithelium. This effect promotes the growth of other commensals that participate in the development of healthy mucosal immunity and provide benefits in gut tissue and beyond [49].
hysical exercise has positive effects on GIT microbiome biodiversity, as observed in several animal and human studies [10, 50, 51]. Several authors suggest that these changes in microbiome diversity are associated with cardiorespiratory fitness and GIT microbial metabolic profile [49, 52, 53]. People with better cardiorespiratory fitness have better microbial diversity and chemotaxis activity with decreased lipopolysaccharide (LPS) biosynthesis [52]. This improvement in microbial diversity and cardiorespiratory fitness in exercising subjects may be attributed to higher abundance of butyrate-producing bacteria by the Clostridiales, Erysipelotrichaceae, Lachnospiraceae, and Roseburia families.
icrobiome involvement in metabolic diseases is perhaps most vividly portrayed in gnotobiotic rodents and probiotic/prebiotic supplementation studies. Allen et al. reported that microbiome transplanted from exercising mice to gnotobiotic mice improved bacterial diversity and metabolite profile and decreased colon inflammation [54]. These changes in microbiome, metabolites, and inflammation markers are linked to gut permeability during scenarios of physiological stress. Therefore, targeting the intestinal microbiota may provide a novel strategy to prevent increases in intestinal permeability and thus to cope with physiological stress [55]. Exercise started in early life is more effective in correcting microbiome (increasing Bacteroidetes to Firmicutes ratio) and in developing stable lean body mass [56]. An increase in Firmicutes to Bacteroidetes ratio, characterized by microbiome enrichment with Firmicutes or depletion of Bacteroidetes, is recognized as an obesogenic trait and is often found in obese children [57]. Furthermore, an increase in butyrate-producing bacteria influences the metabolic pathways involved in fat accumulation and prevents obesity. Estaki et al. reported an increased production of SCFAs and reduced biosynthesis of LPS by gram-negative bacteria in exercising subjects, suggesting a protective role of exercise in immune-inflammatory inhibition of ORMDs [52]. Similarly, Hsu et al. observed that probiotic Bacteroides fragilis supplementation improved exercise tolerance and swimming performance in gnotobiotic mice [58]. The improvement in exercise tolerance was also associated with improvements in liver, muscle, brown fat weight, and the antioxidant enzyme production system [58]. Although the initial response to intense exercise regimes constitutes an increase in gut barrier permeability, continuing exercise for longer duration may improve GIT microbial diversity which attenuates production of the cytokines involved in tissue regeneration [54].
larke et al. reported conformational changes in microbiome diversity in high-performance rugby player's stool, particularly, a decrease in Firmicutes to Bacteroidetes ratio [59], which has implications for the pathogenesis of obesity and diabetes [8]. Data from the American Gut Project revealed that adopting moderate exercise (from never to daily) increases species diversity among Firmicutes phylum and promotes healthier gut environment [60]. Habitual moderate to intense physical activity improves microbial diversity and immune functions and reduces inflammatory diseases [61]. Petersen et al. performed shotgun metagenomics and metatranscriptomic analysis of competitive cyclists, and reported changes in microbial diversity and an upregulation in carbohydrate metabolism and energy production [62]. Similarly, Welly et al. reported that exercise-induced microbiome changes in rats were associated with low insulin resistance, adipose tissue inflammation, and better exercise tolerance [63].
In most of the published studies, exercise-induced microbiome changes are investigated at the holistic level, while very few studies describe specific taxonomic associations between exercise and GIT microbiome. However, a few rodent exercise studies have associated weight loss with an increase in the Bacteroidetes/Firmicutes ratio [64, 65]. Furthermore, investigators observed a positive correlation between Lactobacillus and Bifidobacterium spp. and serum leptin levels in exercising rats. Although we assume that an increase in the Bacteroidetes/Firmicutes ratio is beneficial for metabolic health, as has been observed in several studies [57, 59, 64-66], there are also contradictory findings, suggesting exercise-induced health benefits in association with increased Firmicutes diversity and depletion in Bacteroidetes populations [60, 67]. These differences in observations may be attributed to different exercise interventions, dietary patterns, or different genetic background of the host. The members of the Firmicutes phylum are most responsive to exercise-associated microbiome changes. However, it is hard to identify specific bacteria genera that create beneficial health responses or that lead to systemic inflammation.
Most of the studies reviewed here reported that the changes in microbiome diversity are associated with improvements in health status. Perhaps, most of these studies describe dissimilar taxonomical changes in microbiome in response to exercise, and generally present more global changes at phylum level (e.g. changes in Bacteroidetes/Firmicutes ratio) or in terms of alpha and beta diversity (species richness and diversity). These inconsistent taxonomical observations may be due to different experimental settings, such as differences in subjects' age, sex, disease state, supplementation regime, and exercise pattern. Although, similar exercising patterns may have different results in different ages [56] or diet groups [64], Allen et al. reported that different exercising patterns (voluntary wheel running vs. forced treadmill running) may also affect microbiome differently [68]. Therefore, the only conclusive observations in almost all of these studies are that exercise increases microbial species' richness and evenness (alpha diversity), and results in distinct clustering patterns (beta diversity), and that there is no single microbial taxon or group of bacteria that can be associated with such variation, as reported elsewhere [9, 10, 58].
Furthermore, most of the reviewed studies recapitulate enhanced production of SCFA, particularly butyrate [53, 64, 69], which are produced by microbial fermentation of fibers and non-digestible starch. This discussion endorses a significant role of physical exercise in microbial metabolism and energy cycles. Perhaps, further mechanistic experimentation is required to elucidate host energy homeostasis in the framework of mode and duration of training that imprints positive effects on microbial community and its functional containment. The future goals of research on exercise-induced microbiome changes should include molecular evaluation of immune-inflammatory pathways or neuroendocrine mediators in gut and metabolic tissues.
6. Metabolic disorders in the view of exercise-induced microbial diversity
Patients with metabolic disorders are characterized by excessive release of pro-inflammatory cytokines from bone marrow-derived monocytes, NF-κB, and chemoattractant protein 1 that disturb the blood-brain barrier and induce neuroinflammatory responses [70, 71]. Inactive lifestyle enhances fatigability and acts as mediator of the development of obesity, hypertension, dyslipidemia, and insulin resistance [72]. In a sedentary rat model of metabolic syndrome, Feng et al. observed that exercise rectified immune-inflammatory markers of obesity and immune-inflammatory responses [73]. These changes in ORMD markers were coequally associated with an exercise-induced improvement in microbiome diversity.
Recently, Dalton et al. reviewed the effects of exercise on the gut-brain axis and microbiome diversity [74]. The authors proposed that exercise-induced improvements in mood and psychological disorders are linked with changes in microbial diversity [75]. Increase in microbiome diversity in response to continuous aerobic exercise has been shown to stabilize GIT barrier function and lower symptoms of irritable bowel syndrome [76]. Probiotic supplementation and aerobic exercise may improve the diversity and abundance of genera from the Firmcutes phylum [76]. This may be explained as a neuroendocrine link between the positive effects of exercise on GIT and brain to prevent or treat psychological distress through the microbiome-gut-brain axis [74]. This neuroendocrine containment within the microbiome influences behavior or learning aptitude through biosynthesis of microbial neurochemicals either directly or through bi-directional transfer of genetic information between host and the microbiome [23].
Allen et al. observed that at baseline, the fecal microbiome, microbiome metabolic profile, and cardiorespiratory fitness level of obese subjects were different from those of lean subjects, and exercise training was positively correlated with these parameters [77]. However, when the rats were returned to a sedentary lifestyle, these changes also reverted to baseline values. Furthermore, exercise-induced microbiome changes reduced fatigue and depression, and improved exercise tolerance, motivation, and cardiorespiratory fitness. Similarly, Feng et al. showed that pre-operative exercise minimizes postoperative weight-gain, neuroinflammation, and cognitive decline and corrects the microbiome in rats [78]. Carbajo-Pescador et al. studied the effects of exercise training on the functionality of gut microbiota, intestinal barrier integrity, and hepatic steatosis in high-fat diet rats ]9]. Exercise lowered body weight, mitigated metabolic syndrome and hepatic steatosis, and improved intestinal barrier function.
These exercise-induced metabolic changes were associated with an increased population density of Bacteroides, Flavobacterium, and Parabacteroides genera. These microbial genera have been recognized previously for their protective role against obesity, liver steatosis, and anti-inflammatory capacities [79, 80]. Denou et al. reported that exercise could improve the Bacteroidetes/Firmicutes ratio, metabolic rate, and tricarboxylic acid pathway genes in high-fat diet fed obese mice [66]. In general, overabundance of phylum Firmicutes is associated with obesity, whereas increase in Phylum Bacteriodetes is associated with weight loss in obese individuals. Similarly, higher species richness and diversity of the GIT microbiome corresponds to improved metabolic markers and energy balance, whereas low bacterial diversity is correlated with insulin resistance, obesity, and dyslipidemia (Figure 3) [81]. Since continuous physical exercise is known to prevent or treat these metabolic diseases, these findings may suggest that exercise-associated changes in the GIT microbiome may support the host's metabolic health. Furthermore, enhanced microbiome diversity in endurance performers can also improve antioxidant capacity, which is crucial in lowering insulin resistance and preventing ORMDs [58, 82].
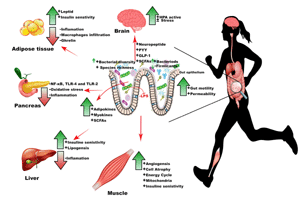 |
 |
Figure 3. How does physical exercise influence the microbiome and how does the microbiome then impact on metabolism?. Physical exercise changes GIT barrier permeability, GIT motility, and microbial diversity, which subsequently change the metabolic profile of liver, adipose tissue, and muscles. An improvement in gut barrier permeability prevents systemic release of lipopolysaccharides (LPS) and inflammation. Furthermore, exercise-induced changes in the microbiome activate the hypothalamus-pituitary-adrenal (HPA) axis and reduce different types of stress. Subsequent release of short-chain fatty acid (SCFAs) and activation of adipokines and chemokines prevent inflammation and autoimmune destruction of beta-cells, and improve energy balance and tissue metabolism. |
|
Another plausible mechanism that may impart exercise-induced changes in the microbiome in ORMDs is the change in microbiome metabolic profile. The microbiota-produced SCFA activate the 5' AMP-activated protein kinase (AMPK) pathway to control lipids and glucose metabolism and act as a sensor for cellular energy status [83]. GIT microbiome is critical for sodium-dependent glucose transporter 1 (SGLT1) and G-protein-coupled receptor (GPR) expression on colon epithelial cells. However, it has been observed that antibiotic-induced GIT microbiota depletion in rats results in impaired muscle function in endurance running performance. Furthermore, decrease in microbial diversity is also associated with loss in muscle glycogen levels and insufficient metabolic performance because of SGLT1 and GPR depletion [84]. However, SCFA-regulated GPR stimulation controls size and function (expression of Foxp3 and IL-10) of the colonic T regulatory (Treg) and T helper (Th) cells in intestinal inflammation [85, 86]. Therefore, a decrease in the richness of bacteria species, as observed during antibiotic therapy, may suppress SCFA production, which eventually results in decreased Treg cell production and activation of pro-inflammatory and autoimmune pathways.
Furthermore, exercise increased fecal abundance of arabinose and carnosine metabolites, which are promising candidate for therapies to combat diabetes and obesity [87]. These microbes and metabolites prevent LPS influx, TLR-4-mediated NF-κB activation, and inflammatory cytokine production. Eventually, downregulation of TLR-2, TLR-4, and the NF-κB pathway resulting from exercise may improve insulin sensitivity [88]. In brief, exercise-induced changes in GIT microbiome and metabolome help to maintain intestine wall integrity and downregulate both local and systemic immune-inflammatory pathways that may contribute to the prevention of ORMDs [51].
As discussed in the above sections and shown in Table 1, most of the literature available provides only common diversity or phylum level taxonomic changes in microbiome in response to exercise, both in health and metabolic diseases. It is therefore reasonable to assume that exercise-induced changes in microbiome diversity could improve host metabolic profile by:
- Decreasing lipid production and storage in visceral organs
- Correcting chronic low-grade inflammation
- Decreasing insulin resistance
Table
1.
Summary of exercise-induced gut microbiome changes observed in different metabolic syndrome conditions |
|
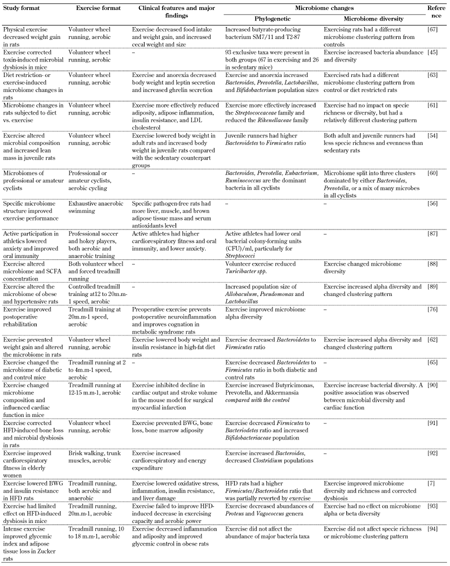 |
 |
Legend:
BWG - body weight gain, CFU - colony forming unit, HFD - high-fat diet, LDL - low-density lipoprotein, SCFA - short-chain fatty acid. References: [7, 45, 54, 56, 60-63, 65, 67, 76, 87-94]. |
|
We have attempted to establish a link between exercise-induced phylogenetic changes in microbiome and metabolic disorders that associate microbiome changes with predictive immune-inflammatory pathways. Eventually, this may provide a mechanistic understanding of the beneficial effects of physical exercise in metabolic disorders through microbiome changes.
7. Conclusions
The observations reviewed here suggest a plausible microbiome basis for exercise-induced prevention of metabolic diseases, and provide an opportunity to explore novel avenues to prevent metabolic diseases in critically lethargic subjects who are incapable of voluntarily exercise to manage their weight. Although, most of the favorable health outcomes are independent of microbiome-host interactions, there is also evidence to indicate that some of the benefits may be mediated by exercise-induced adaptations in gut microorganisms.
Reciprocal associations between immune-inflammatory mediators and exercise-induced microbiome/metabolome corrections may engage several cellular energy sensors and trigger adipokine and myokine activation that monitor energy balance and tissue metabolism. Although abundant evidence is available on the role of the microbiome in metabolic disorders, no significant clinical findings are available to prevent or correct these disorders. Extensive work is needed on metagenomics beyond taxonomic diversity profiling. More mechanistic research is required to connect exercise with microbiome, body metabolism, and energy homeostasis.
Author contributions: MUS wrote the manuscript, AS prepared the diagrams, and AA and HMY reviewed the manuscript.
Disclosures: The authors report no conflict of interests.
References
- Murray CJ, Lopez AD. Alternative projections of mortality and disability by cause 1990-2020: Global Burden of Disease Study. The Lancet 1997. 349(9064):1498-1504. [DOD] [CrossRef]
- Shabbir MZ, Mustafa I, Sohaile MU. Shiitake culinary-medicinal mushroom, lentinus edodes (agaricomycetes), supplementation alters gut microbiome and corrects dyslipidemia in rats. Int J Med Mushr 2019. 21(1):79-88. [DOD] [CrossRef]
- Ussar S, Fujisaka S, Kahn CR. Interactions between host genetics and gut microbiome in diabetes and metabolic syndrome. Mol Metab 2016. 5(9):795-803. [DOD] [CrossRef]
- Lee SA, Lim JY, Kim BS, Cho SJ, Kim NY, Kim OB, Kim Y. Comparison of the gut microbiota profile in breast-fed and formula-fed Korean infants using pyrosequencing. Nutr Res Pract 2015. 9(3):242-248. [DOD] [CrossRef]
- West CE, Renz H, Jenmalm MC, Kozyrskyj AL, Allen KJ, Vuillermin P, Prescott SL. The gut microbiota and inflammatory noncommunicable diseases: associations and potentials for gut microbiota therapies. J Allergy Clin Immunol 2015. 135(1):3-13. [DOD] [CrossRef]
- Barton W, Penney NC, Cronin O, Garcia-Perez I, Molloy MG, Holmes E, Shanahan F, Cotter PD, O'Sullivan O. The microbiome of professional athletes differs from that of more sedentary subjects in composition and particularly at the functional metabolic level. Gut 2018. 67(4):625-633. [DOD]
- Sohail MU, Shabbir MZ, Steiner JM, Ahmad S, Kamran Z, Anwar H, Hussain G, Shaukat A, Suchodolski JS. Molecular analysis of the gut microbiome of diabetic rats supplemented with prebiotic, probiotic, and synbiotic foods. Int J Diabetes Dev Countr 2016. 2016:1-7. [DOD]
- Sohail MU, Althani A, Anwar H, Rizzi R, Marei HE. Role of the gastrointestinal tract microbiome in the pathophysiology of diabetes mellitus. J Diabetes Res 2017. 2017:9631435. [DOD] [CrossRef]
- Carbajo-Pescador S, Porras D, Garcia-Mediavilla MV, Martinez-Florez S, Juarez-Fernandez M, Cuevas MJ, Mauriz JL, Gonzalez-Gallego J, Nistal E, Sanchez-Campos S. Beneficial effects of exercise on gut microbiota functionality and barrier integrity, and gut-liver crosstalk in an in vivo model of early obesity and non-alcoholic fatty liver disease. Dis Model Mechan 2019. 12(5):dmm039206. [DOD] [CrossRef]
- Clarke SF, Murphy EF, O'Sullivan O, Lucey AJ, Humphreys M, Hogan A, Hayes P, O'Reilly M, Jeffery IB, Wood-Martin R. Exercise and associated dietary extremes impact on gut microbial diversity. Gut 2014. 63(12):1913-1920. [DOD] [CrossRef]
- Abbott A. Scientists bust myth that our bodies have more bacteria than human cells. Nature 2016. 2016:19136. [DOD]
- Bäckhed F, Roswall J, Peng Y, Feng Q, Jia H, Kovatcheva-Datchary P, Li Y, Xia Y, Xie H, Zhong H. Dynamics and stabilization of the human gut microbiome during the first year of life. Cell Host Microbe 2015. 17(5):690-703. [DOD] [CrossRef]
- Maurice CF, Haiser HJ, Turnbaugh PJ. Xenobiotics shape the physiology and gene expression of the active human gut microbiome. Cell 2013. 152(1):39-50. [DOD] [CrossRef]
- Zhernakova A, Kurilshikov A, Bonder MJ, Tigchelaar EF, Schirmer M, Vatanen T, Mujagic Z, Vila AV, Falony G, Vieira-Silva S, et al. Population-based metagenomics analysis reveals markers for gut microbiome composition and diversity. Science 2016. 352(6285):565-569. [DOD] [CrossRef]
- Neish AS. Mucosal immunity and the microbiome. Ann Am Thorac Soc 2014. 11(Supplement 1):S28-S32. [DOD] [CrossRef]
- Trompette A, Gollwitzer ES, Yadava K, Sichelstiel AK, Sprenger N, Ngom-Bru C, Blanchard C, Junt T, Nicod LP, Harris NL. Gut microbiota metabolism of dietary fiber influences allergic airway disease and hematopoiesis. Nat Med 2014. 20(2):159. [DOD] [CrossRef]
- Cani PD, Knauf C. How gut microbes talk to organs: the role of endocrine and nervous routes. Mol Metab 2016. 5(9):743-752. [DOD] [CrossRef]
- Ridaura V, Belkaid Y. Gut microbiota: the link to your second brain. Cell 2015. 161(2):193-194. [DOD] [CrossRef]
- Barrett E, Ross R, O'Toole P, Fitzgerald G, Stanton C. Gamma-aminobutyric acid production by culturable bacteria from the human intestine. J Appl Microbiol 2012. 113(2):411-417. [DOD] [CrossRef]
- Clarke G, Stilling RM, Kennedy PJ, Stanton C, Cryan JF, Dinan TG. Minireview: gut microbiota: the neglected endocrine organ. Mol Endocrinol 2014. 28(8):1221-1238. [DOD] [CrossRef]
- Sohail M, Ijaz A, Yousaf M, Ashraf K, Zaneb H, Aleem M, Rehman H. Alleviation of cyclic heat stress in broilers by dietary supplementation of mannan-oligosaccharide and Lactobacillus-based probiotic: Dynamics of cortisol, thyroid hormones, cholesterol, C-reactive protein, and humoral immunity. Poult Sci 2010. 89(9):1934-1938. [DOD] [CrossRef]
- Sudo N, Chida Y, Aiba Y, Sonoda J, Oyama N, Yu XN, Kubo C, Koga Y. Postnatal microbial colonization programs the hypothalamic–pituitary–adrenal system for stress response in mice. J Physiol 2004. 558(1):263-275. [DOD] [CrossRef]
- Cryan JF, Dinan TG. Mind-altering microorganisms: the impact of the gut microbiota on brain and behaviour. Nature Rev Neurosci 2012. 13(10):701-712. [DOD] [CrossRef]
- Byrne C, Chambers E, Morrison D, Frost G. The role of short chain fatty acids in appetite regulation and energy homeostasis. Int J Obes 2015. 39(9):1331. [DOD] [CrossRef]
- Ang Z, Er JZ, Ding JL. The short-chain fatty acid receptor GPR43 is transcriptionally regulated by XBP1 in human monocytes. Sci Rep 2015. 5:8134. [DOD] [CrossRef]
- D'Souza WN, Douangpanya J, Mu S, Jaeckel P, Zhang M, Maxwell JR, Rottman JB, Labitzke K, Willee A, Beckmann H. Differing roles for short chain fatty acids and GPR43 agonism in the regulation of intestinal barrier function and immune responses. Plos One 2017. 12(7):e0180190. [DOD] [CrossRef]
- Fothergill E, Guo J, Howard L, Kerns JC, Knuth ND, Brychta R, Chen KY, Skarulis MC, Walter M, Walter PJ. Persistent metabolic adaptation 6 years after “The Biggest Loser” competition. Obesity 2016. 24(8):1612-1619. [DOD] [CrossRef]
- Grundlingh J, Dargan PI, El-Zanfaly M, Wood DM. 2,4-dinitrophenol (DNP): a weight loss agent with significant acute toxicity and risk of death. J Med Toxicol 2011. 7(3):205. [DOD] [CrossRef]
- Hassan Y, Head V, Jacob D, Bachmann M, Diu S, Ford J. Lifestyle interventions for weight loss in adults with severe obesity: a systematic review. Clin Obes 2016. 6(6):395-403. [DOD] [CrossRef]
- Thaiss CA, Itav S, Rothschild D, Meijer MT, Levy M, Moresi C, Dohnalova L, Braverman S, Rozin S, Malitsky S. Persistent microbiome alterations modulate the rate of post-dieting weight regain. Nature 2016. 540(7634):544. [DOD] [CrossRef]
- Palleja A, Kashani A, Allin KH, Nielsen T, Zhang C, Li Y, Brach T, Liang S, Feng Q, Jorgensen NB. Roux-en-Y gastric bypass surgery of morbidly obese patients induces swift and persistent changes of the individual gut microbiota. Genome Med 2016. 8(1):67. [DOD] [CrossRef]
- Kootte R, Vrieze A, Holleman F, Dallinga‐Thie GM, Zoetendal EG, de Vos WM, Groen A, Hoekstra JB, Stroes ES, Nieuwdorp M. The therapeutic potential of manipulating gut microbiota in obesity and type 2 diabetes mellitus. Diabetes Obes Metab 2012. 14(2):112-120. [DOD] [CrossRef]
- Brown K, Godovannyi A, Ma C, Zhang Y, Ahmadi-Vand Z, Dai C, Gorzelak MA, Chan Y, Chan JM, Lochner A. Prolonged antibiotic treatment induces a diabetogenic intestinal microbiome that accelerates diabetes in NOD mice. ISME J 2016. 10(2):321. [DOD] [CrossRef]
- Cho I, Yamanishi S, Cox L, Methe BA, Zavadil J, Li K, Gao Z, Mahana D, Raju K, Teitler I. Antibiotics in early life alter the murine colonic microbiome and adiposity. Nature 2012. 488(7413):621. [DOD] [CrossRef]
- Hicks LA, Bartoces MG, Roberts RM, Suda KJ, Hunkler RJ, Taylor TH Jr, Schrag SJ. US outpatient antibiotic prescribing variation according to geography, patient population, and provider specialty in 2011. Clin Infect Dis 2015. 60(9):1308-1316. [DOD]
- Mottawea W, Chiang CK, Mühlbauer M, Starr AE, Butcher J, Abujamel T, Deeke SA, Brandel A, Zhou H, Shokralla S. Altered intestinal microbiota-host mitochondria crosstalk in new onset Crohn's disease. Nature Commun 2016. 7:13419. [DOD] [CrossRef]
- Bäckhed F, Ding H, Wang T, Hooper LV, Koh GY, Nagy A, Semenkovich CF, Gordon JI. The gut microbiota as an environmental factor that regulates fat storage. Proc Nat Acad Sci 2004. 101(44):15718-15723. [DOD] [CrossRef]
- Turnbaugh PJ, Ley RE, Mahowald MA, Magrini V, Mardis ER, Gordon JI. An obesity-associated gut microbiome with increased capacity for energy harvest. Nature 2006. 444(7122):1027. [DOD] [CrossRef]
- Cani PD, Van Hul M, Lefort C, Depommier C, Rastelli M, Everard A. Microbial regulation of organismal energy homeostasis. Nature Metab 2019. 1(1):34. [DOD] [CrossRef]
- Rosenbaum M, Knight R, Leibel RL. The gut microbiota in human energy homeostasis and obesity. Trends Endocrinol Metab 2015. 26(9):493-501. [DOD] [CrossRef]
- Lowder T, Padgett DA, Woods JA. Moderate exercise early after influenza virus infection reduces the Th1 inflammatory response in lungs of mice. Exerc Immunol Rev 2006. 12:97-111. [DOD]
- Hoffman-Goetz L, Pervaiz N, Packer N, Guan J. Freewheel training decreases pro-and increases anti-inflammatory cytokine expression in mouse intestinal lymphocytes. Brain Behav Immun 2010. 24(7):1105-1115. [DOD] [CrossRef]
- Hoffman-Goetz L, Quadrilatero J. Treadmill exercise in mice increases intestinal lymphocyte loss via apoptosis. Acta Physiol 2003. 179(3):289-297. [DOD] [CrossRef]
- Marchesi JR, Adams DH, Fava F, Hermes GD, Hirschfield GM, Hold G, Quraishi MN, Kinross J, Smidt H, Tuohy KM. The gut microbiota and host health: a new clinical frontier. Gut 2016. 65(2):330-339. [DOD] [CrossRef]
- Dokladny K, Zuhl MN, Moseley PL. Intestinal epithelial barrier function and tight junction proteins with heat and exercise. J Appl Physiol 2015. 120(6):692-701. [DOD] [CrossRef]
- Zuhl M, Dokladny K, Mermier C, Schneider S, Salgado R, Moseley P. The effects of acute oral glutamine supplementation on exercise-induced gastrointestinal permeability and heat shock protein expression in peripheral blood mononuclear cells. Cell Stress Chaperones 2015. 20(1):85-93. [DOD] [CrossRef]
- Choi JJ, Eum SY, Rampersaud E, Daunert S, Abreu MT, Toborek M. Exercise attenuates PCB-induced changes in the mouse gut microbiome. Environ Health Perspect 2013. 121(6):725-730. [DOD] [CrossRef]
- Estaki M, Pither J, Baumeister P, Little J, Gill S, Ghosh S, Marsden K, Ahmadi-Vand Z, Gibson D. Physical activity as a modulator of intestinal Microbiota, immune response, and short-chain fatty acids production. J Canad Assoc Gastroenterol 2018. 1(Suppl 1):497-498. [DOD] [CrossRef]
- Mach N, Fuster-Botella D. Endurance exercise and gut microbiota: a review. J Sport Health Sci 2017. 6(2):179-197. [DOD] [CrossRef]
- Batacan R, Fenning A, Dalbo V, Scanlan A, Duncan M, Moore R, Stanley D. A gut reaction: the combined influence of exercise and diet on gastrointestinal microbiota in rats. J Appl Microbiol 2017. 122(6):1627-1638. [DOD] [CrossRef]
- Campbell SC, Wisniewski PJ, Noji M, McGuinness LR, Häggblom MM, Lightfoot SA, Joseph LB, Kerkhof LJ. The effect of diet and exercise on intestinal integrity and microbial diversity in mice. Plos One 2016. 11(3):e0150502. [DOD] [CrossRef]
- Estaki M, Pither J, Baumeister P, Little JP, Gill SK, Ghosh S, Ahmadi-Vand Z, Marsden KR, Gibson DL. Cardiorespiratory fitness as a predictor of intestinal microbial diversity and distinct metagenomic functions. Microbiome 2016. 4(1):42. [DOD] [CrossRef]
- Kang SS, Jeraldo PR, Kurti A, Miller MEB, Cook MD, Whitlock K, Goldenfeld N, Woods JA, White BA, Chia N. Diet and exercise orthogonally alter the gut microbiome and reveal independent associations with anxiety and cognition. Molecul Neurodegen 2014. 9(1):36. [DOD] [CrossRef]
- Allen J, Mailing L, Cohrs J, Salmonson C, Fryer J, Nehra V, Hale V, Kashyap P, White B, Woods J. Exercise training-induced modification of the gut microbiota persists after microbiota colonization and attenuates the response to chemically-induced colitis in gnotobiotic mice. Gut Microbes 2017. 2017:1-16. [DOD]
- Karl JP, Margolis LM, Madslien EH, Murphy NE, Castellani JW, Gundersen Y, Hoke AV, Levangie MW, Kumar R, Chakraborty N. Changes in intestinal microbiota composition and metabolism coincide with increased intestinal permeability in young adults under prolonged physiological stress. Am J Physiol Gastrointest Liver Physiol 2017. 312(6):G559-G571. [DOD] [CrossRef]
- Mika A, Van Treuren W, Gonzalez A, Herrera JJ, Knight R, Fleshner M. Exercise is more effective at altering gut microbial composition and producing stable changes in lean mass in juvenile versus adult male F344 rats. Plos One 2015. 10(5):e0125889. [DOD] [CrossRef]
- Riva A, Borgo F, Lassandro C, Verduci E, Morace G, Borghi E, Berry D. Pediatric obesity is associated with an altered gut microbiota and discordant shifts in Firmicutes populations. Environ Microbiol 2017. 19(1):95-105. [DOD] [CrossRef]
- Hsu YJ, Chiu CC, Li YP, Huang WC, Te Huang Y, Huang CC, Chuang HL. Effect of intestinal microbiota on exercise performance in mice. J Strength Condition Res 2015. 29(2):552-558. [DOD] [CrossRef]
- Clarke SF, Murphy EF, O'Sullivan O, Lucey AJ, Humphreys M, Hogan A, Hayes P, O'Reilly M, Jeffery IB, Wood-Martin R. Exercise and associated dietary extremes impact on gut microbial diversity. Gut 2014. 63(12):1913-1920. [DOD] [CrossRef]
- McFadzean R. Exercise can help modulate human gut microbiota. 2014. Thesis. [DOD]
- Walsh NP, Gleeson M, Shephard RJ, Gleeson M, Woods JA, Bishop N, Fleshner M, Green C, Pedersen BK, Hoffman-Goete L. Position statement part one: immune function and exercise. Exerc Immunol Rev 2011. 17:6-63. [DOD]
- Petersen LM, Bautista EJ, Nguyen H, Hanson BM, Chen L, Lek SH, Sodergren E, Weinstock GM. Community characteristics of the gut microbiomes of competitive cyclists. Microbiome 2017. 5(1):98. [DOD] [CrossRef]
- Welly RJ, Liu TW, Zidon TM, Rowles III JL, Park YM, Smith TN, Swanson KS, Padilla J, Vieira-Potter VJ. Comparison of diet vs. exercise on metabolic function and gut microbiota in obese rats. Med Sci Sports Exerc 2016. 48(9):1688-1698. [DOD] [CrossRef]
- Evans CC, LePard KJ, Kwak JW, Stancukas MC, Laskowski S, Dougherty J, Moulton L, Glawe A, Wang Y, Leone V. Exercise prevents weight gain and alters the gut microbiota in a mouse model of high fat diet-induced obesity. Plos One 2014. 9(3):e92193. [DOD] [CrossRef]
- Queipo-Ortuno MI, Seoane LM, Murri M, Pardo M, Gomez-Zumaquero JM, Cardona F, Casanueva F, Tinahones FJ. Gut microbiota composition in male rat models under different nutritional status and physical activity and its association with serum leptin and ghrelin levels. Plos One 2013. 8(5):e65465. [DOD] [CrossRef]
- Denou E, Marcinko K, Surette MG, Steinberg GR, Schertzer JD. High-intensity exercise training increases the diversity and metabolic capacity of the mouse distal gut microbiota during diet-induced obesity. Am J Physiol Endocrinol Metab 2016. 310(11):E982-E993. [DOD] [CrossRef]
- Lambert JE, Myslicki JP, Bomhof MR, Belke DD, Shearer J, Reimer RA. Exercise training modifies gut microbiota in normal and diabetic mice. Appl Physiol Nutr Metab 2015. 40(7):749-752. [DOD] [CrossRef]
- Allen JM, Berg Miller ME, Pence BD, Whitlock K, Nehra V, Gaskins HR, White BA, Fryer JD, Woods JA. Voluntary and forced exercise differentially alters the gut microbiome in C57BL/6J mice. J Appl Physiol 2015. 118(8):1059-1066. [DOD] [CrossRef]
- Matsumoto M, Inoue R, Tsukahara T, Ushida K, Chiji H, Matsubara N, Hara H. Voluntary running exercise alters microbiota composition and increases n-butyrate concentration in the rat cecum. Biosci Biotechnol Biochem 2008. 72(2):572-576. [DOD] [CrossRef]
- Terrando N, Eriksson LI, Kyu Ryu J, Yang T, Monaco C, Feldmann M, Jonsson Fagerlund M, Charo IF, Akassoglou K, Maze M. Resolving postoperative neuroinflammation and cognitive decline. Ann Neurol 2011. 70(6):986-995. [DOD] [CrossRef]
- Vacas S, Degos V, Tracey KJ, Maze M. High-mobility group box 1 protein initiates postoperative cognitive decline by engaging bone marrow-derived macrophages. Anesthesiology 2014. 120(5):1160-1167. [DOD] [CrossRef]
- Sisson SB, Camhi SM, Church TS, Martin CK, Tudor-Locke C, Bouchard C, Earnest CP, Smith SR, Newton M Jr, Robert L, Rankinen T. Leisure time sedentary behavior, occupational/domestic physical activity, and metabolic syndrome in US men and women. Metab Syndr Rel Disord 2009. 7(6):529-536. [DOD] [CrossRef]
- Feng X, Uchida Y, Koch L, Britton S, Hu J, Lutrin D, Maze M. Exercise prevents enhanced postoperative neuroinflammation and cognitive decline and rectifies the gut microbiome in a rat model of metabolic syndrome. Front Immunol 2017. 8:1768. [DOD] [CrossRef]
- Dalton A, Mermier C, Zuhl M. Exercise influence on the microbiome-gut-brain axis. Gut Microbes 2019. 2019:1-14. [DOD]
- Liu HN, Wu H, Chen YZ, Chen YJ, Shen XZ, Liu TT. Altered molecular signature of intestinal microbiota in irritable bowel syndrome patients compared with healthy controls: a systematic review and meta-analysis. Digest Liver Dis 2017. 49(4):331-337. [DOD] [CrossRef]
- Clark A, Mach N. Exercise-induced stress behavior, gut-microbiota-brain axis and diet: a systematic review for athletes. J Int Soc Sports Nutr 2016. 13(1):43. [DOD] [CrossRef]
- Allen JM, Mailing LJ, Niemiro GM, Moore R, Cook MD, White BA, Holscher HD, Woods JA. Exercise alters gut microbiota composition and function in lean and obese humans. Urbana 2017. 51:61801. [DOD]
- Feng X, Uchida Y, Koch L, Britton S, Hu J, Lutrin D, Maze M. Exercise prevents enhanced Postoperative neuroinflammation and cognitive Decline and rectifies the gut microbiome in a rat model of metabolic syndrome. Front Immunol 2017. 8:1768. [DOD] [CrossRef]
- Porras D, Nistal E, Martinez-Florez S, Olcoz JL, Jover R, Jorquera F, Gonzalez-Gallego J, Garcia-Mediavilla MV, Sanchez-Campos S. Functional interactions between gut microbiota transplantation, quercetin, and high-fat diet determine non-alcoholic fatty liver disease development in germ-free mice. Mol Nutr Food Res 2019. 2019:1800930. [DOD]
- Porras D, Nistal E, Martinez-Florez S, Pisonero-Vaquero S, Olcoz JL, Jover R, Gonzalez-Gallego J, Garcia-Mediavilla MV, Sanchez-Campos S. Protective effect of quercetin on high-fat diet-induced non-alcoholic fatty liver disease in mice is mediated by modulating intestinal microbiota imbalance and related gut-liver axis activation. Free Radic Biol Med 2017. 102:188-202. [DOD] [CrossRef]
- Foley KP, Zlitni S, Denou E, Duggan BM, Chan RW, Stearns JC, Schertzer JD. Long term but not short term exposure to obesity related microbiota promotes host insulin resistance. Nature Commun 2018. 9(1):4681. [DOD] [CrossRef]
- Tangvarasittichai S. Oxidative stress, insulin resistance, dyslipidemia and type 2 diabetes mellitus. World J Diabetes 2015. 6(3):456. [DOD] [CrossRef]
- den Besten G, van Eunen K, Groen AK, Venema K, Reijngoud DJ, Bakker BM. The role of short-chain fatty acids in the interplay between diet, gut microbiota, and host energy metabolism. J Lipid Res 2013. 54(9):2325-2340. [DOD] [CrossRef]
- Nay K, Jollet M, Goustard B, Baati N, Vernus B, Pontones M, Lefeuvre-Orfila L, Bendavid C, Rué O, Mariadassou M. Gut bacteria are critical for optimal muscle function: a potential link with glucose homeostasis. Am J Physiol Endocrinol Metab 2019. In press. [DOD]
- Mamontov P, Neiman E, Cao T, Perrigoue J, Friedman J, Das A, Mora J. Effects of short chain fatty acids and GPR43 stimulation on human Treg function (IRC5P. 631). J Immunol 2015. 194:58. [DOD]
- Smith PM, Howitt MR, Panikov N, Michaud M, Gallini CA, Bohlooly-Y M, Glickman JN, Garrett WS. The microbial metabolites, short-chain fatty acids, regulate colonic Treg cell homeostasis. Science 2013. 341(6145):569-573. [DOD] [CrossRef]
- Schaalan MF, Ramadan BK, Abd Elwahab AH. Synergistic effect of carnosine on browning of adipose tissue in exercised obese rats; a focus on circulating irisin levels. J Cell Physiol 2018. 233(6):5044-5057. [DOD] [CrossRef]
- Rada I, Deldicque L, Francaux M, Zbinden-Foncea H. Toll like receptor expression induced by exercise in obesity and metabolic syndrome: a systematic review. Exerc Immunol Rev 2018. 24:60-71. [DOD]
- Lamb AL, Hess DE, Edenborn S, Ubinger E, Carrillo AE, Appasamy PM. Elevated salivary IgA, decreased anxiety, and an altered oral microbiota are associated with active participation on an undergraduate athletic team. Physiol Behav 2017. 169:169-177. [DOD] [CrossRef]
- Woods J, Allen J, Miller MB, White B, Gaskins H, Nehra V. Exercise alters the gut microbiome and microbial metabolites: implications for colorectal cancer and inflammatory bowel disease. Brain Behav Immun 2015. 49:e7. [DOD] [CrossRef]
- Petriz BA, Castro AP, Almeida JA, Gomes CP, Fernandes GR, Kruger RH, Pereira RW, Franco OL. Exercise induction of gut microbiota modifications in obese, non-obese and hypertensive rats. BMC Genom 2014. 15(1):511. [DOD] [CrossRef]
- Liu Z, Liu HY, Zhou H, Zhan Q, Lai W, Zeng Q, Ren H, Xu D. Moderate-intensity exercise affects gut microbiome composition and influences cardiac function in myocardial infarction mice. Front Microbiol 2017. 8:1687. [DOD] [CrossRef]
- McCabe LR, Irwin R, Tekalur A, Evans C, Schepper JD, Parameswaran N, Ciancio M. Exercise prevents high fat diet-induced bone loss, marrow adiposity and dysbiosis in male mice. Bone 2019. 118:20-31. [DOD] [CrossRef]
- Morita E, Yokoyama H, Imai D, Takeda R, Ota A, Kawai E, Hisada T, Emoto M, Suzuki Y, Okazaki K. Aerobic exercise training with brisk walking increases intestinal bacteroides in healthy elderly women. Nutrients 2019. 11(4):868. [DOD] [CrossRef]
|