Review
Rev Diabet Stud,
2010,
7(2):132-143 |
DOI 10.1900/RDS.2010.7.132 |
Mesenchymal Stem Cells as Feeder Cells for Pancreatic Islet Transplants
Valeria Sordi, Lorenzo Piemonti
San Raffaele Diabetes Research Institute (HSR-DRI), Division of Immunology, Transplantation and Infectious Disease, San Raffaele Scientific Institute, Via Olgettina 60, 20132 Milan, Italy
Address correspondence to: Valeria Sordi, e-mail: sordi.valeria@hsr.it
Manuscript submitted July 20, 2010; resubmitted August 7, 2010; accepted August 9, 2010.
Keywords: stem cell, feeder, islet, co-transplantation, revascularization, bone marrow, immunomodulation
Abstract
Allogeneic islet transplantation serves as a source of insulin-secreting beta-cells for the maintenance of normal glucose levels and treatment of diabetes. However, limited availability of islets, high rates of islet graft failure, and the need for life-long non-specific immunosuppressive therapy are major obstacles to the widespread application of this therapeutic approach. To overcome these problems, pancreatic islet transplantation was recently suggested as a potential target of the "therapeutic plasticity" of adult stem cells. In fact, new results suggest that stem/precursor cells, and mesenchymal stem cells in particular, co-transplanted with islets can promote tissue engraftment and beta-cell survival via bystander mechanisms, mainly exerted by creating a milieu of cytoprotective and immunomodulatory molecules. This evidence consistently challenges the limited view that stem/precursor cells work exclusively through beta-cell replacement in diabetes therapy. It proposes that stem cells also act as "feeder" cells for islets, and supporter of graft protection, tissue revascularization, and immune acceptance. This article reviews the experience of using stem cell co-transplantation as strategy to improve islet transplantation. It highlights that comprehension of the mechanisms involved will help to identify new molecular targets and promote development of new pharmacological strategies to treat type 1 and type 2 diabetes patients.
Abbreviations: Balb/c - albino mouse strain (used for the production of monoclonal antibodies); BM - bone marrow; CD4 - cluster of differentiation 4 (glycoprotein expressed on lymphocytes); CD25 - cluster of differentiation 25 (glycoprotein expressed on activated regulatory T cells); CD31 - cluster of differentiation 31 (expressed on neutrophils and macrophages, also severs as endothelial marker); CD34 - cluster of differentiation 34 (glycoprotein, cell-cell adhesion factor, expressed by stem cells); C57BL/6 - inbred mouse strain C57 black 6 (Th1/Th2 immunological response to the same pathogen often opposite to BALBb/c mice); CNS - central nervous system; DC - dendritic cell; EPC - endothelial progenitor cell; FoxP3 - forkhead box P3 (transcriptional regulator protein; marker of natural T regulatory cells); GAD65 - 65 kDa isoform of glutamic acid decarboxylase; HGF - hepatocyte growth factor; HSC - hematopoietic stem cells; IA-2 - islet cell antigen 2 (also called tyrosine phosphatase-like protein); IFN-gamma - interferon gamma; IL-6 - interleukin 6; IL-10 - interleukin 10; MMP - matrix metalloproteinases; MMP2 - matrix metalloproteinase 2 (also known as matrix metallopeptidase 2, gelatinase A, 72kDa gelatinase, and 72kDa type IV collagenase; degrades proteins in the extracellular matrix, proteolytically digests denatured collagen); MMP9 - matrix metalloproteinases 9 (also known as matrix metallopeptidase 9, gelatinase B, 92kDa gelatinase, and 92kDa type IV collagenase; degrades proteins in the extracellular matrix, proteolytically digests denatured collagen); MSC - mesenchymal stem cells; NCSC - neural crest stem cells; NK cell - natural killer cell; NOD - nonobese diabetic; NPC - neural progenitor cell; POD - postoperative day; SCID - severe combined immunodeficiency (mouse model without functional T and B cells); STZ - streptozotocin; TGF-alpha - transforming growth factor alpha; TGF-beta - transforming growth factor beta; VEGF A - vascular endothelial growth factors A
Introduction
Currently, the only clinical therapy capable of restoring beta-cell mass in diabetic patients is the allogenic/autologous transplantation of beta-cells (somatic cell therapy with pancreas, Langherans islets, or beta-cell transplantation). Despite advances in recent years, allogeneic somatic therapy is still problematic. Immunosuppression therapy is necessary, and in case of islet transplantation, many donors are needed for a single recipient. Also, such transplantation therapies have a short life, i.e. patients become diabetic again within a few years of transplantation. These limitations have led to increasing interest in alternative strategies. Several investigations have pursued the generation of insulin-producing cells from other than the primary source (i.e., pancreatic beta-cells). They showed that insulin-producing cells can also be derived from embryonic, adult, mesenchymal stem cells (MSC), and hematopoietic stem cells (HSC) via processes of proliferation, dedifferentiation, neogenesis, nuclear reprogramming, and transdifferentiation.
More recently, the potential role of stem cells in beta-cell regeneration has been reassessed from a different point of view. Whilst efficient and stable maintenance of direct differentiation is very unlikely to be achieved, experimental data suggest that adult stem cells, in particular those of mesodermal origin (i.e. HSC, MSC), are capable of facilitating the survival or endogenous regeneration of beta-cells. Although the mechanism is not yet well-defined. This is an extension of the idea of "therapeutic plasticity" of adult stem cell. The therapeutic plasticity can be viewed as "the capacity of somatic stem cells to adapt their fate and function(s) to specific environmental needs occurring as a result of different pathological conditions". This concept was first described for neural progenitor cells (NPC) [1]. Until recently, it was assumed that the replacement of lost or damaged cells is the prime therapeutic mechanism of NPC. However, it is now clear that transplanted NPC exert immune-like functions, including a "bystander" anti-inflammatory effect that is able to promote central nervous system (CNS) repair. This observation supports the concept that the “therapeutic plasticity”, and in particular the immunomodulatory activity, is a true functional signature of NPC. Nonetheless, it has been demonstrated recently that other sources of somatic stem cells (in particular MSC and HSC), with very low capabilities of neural (trans)differentiation, may show equally significant bystander capacities, and promote CNS repair. This further proves and generalizes the relevance of somatic stem cell-dependent alternative therapeutic mechanisms.
Islet transplantation: an opportunity for "therapeutic plasticity" of adult stem cells
Despite the advent of new insulin preparations and improved methods to monitor glycemia, exogenous insulin administration cannot avoid long-term complications of diabetes. As such, it is not surprising that the life expectancy of diabetic patients is still shorter than that of the general population [2, 3]. Treatment of type 1 and many cases of type 2 diabetes relies on the possibility of finding a beta-cell mass substitute capable of performing two essential functions: assessing blood sugar levels, and secreting appropriate levels of insulin in the vascular bed. Currently, the only available clinical therapies capable of restoring beta-cell mass in diabetic patients are pancreas and islet cell transplantation.
Since the breakthrough made by Shapiro et al. (Edmonton Protocol), islet transplantation has emerged as an attractive alternative to whole pancreas transplantation [4]. In fact, islet transplantation, in contrast to pancreas transplantation, is a much simpler procedure. Islets are obtained from the digestion of pancreas from cadaveric donors, and transplantation occurs by infusion of islets via microembolization into the hepatic portal venous system [5, 6]. This procedure involves risk, but its related morbidity is much less than that of whole pancreas transplantation. The rate of insulin independence at 1 year is ~70%, with virtually all patients maintaining a functioning graft (positive C-peptide), while under adequate immunosuppression levels [5, 7-11]. Overall, sustained graft survival is achieved in the majority of islet transplant recipients, with >70% of them retaining C-peptide levels, normalized glycosylated hemoglobin, and significantly reduced insulin requirements after 5 years [7, 12].
Despite advances in recent years [8], allogeneic islet transplantation is still problematic. Insulin secretion capacity of transplanted recipients is only at ~20-40% of that of healthy persons, even if islets are obtained from 2 to 4 donors. In some studies, as many as 8 to 12 donors were used. Thus, it is apparent that this procedure suffers from low efficacy. The rate of insulin independence declines progressively after transplantation, and may reach ~10% after 5 years, despite maintaining islet graft function [12]. In practical terms, two to three donors are necessary to obtain the minimal cell mass sufficient for successful transplantation into a single recipient (i.e., ~10,000-14,000 islet equivalents per kilogram of recipient body weight) [13]. Thus, the approach is limited by the scarcity of donor organs.
Several factors may contribute to progressive islet graft dysfunction and the observed failure over time. Intrahepatic islet infusion is associated with an immediate blood-mediated inflammatory reaction, thrombosis, and hepatic tissue ischemia with elevated blood liver enzymes. Loss of as many as 50-75% of islets during engraftment has been found the primary factor, necessitating the very large number of islets to achieve normoglycemia. Non-specific immune response mediated predominantly by innate inflammatory processes related to mechanics and site, and pre-existing and transplant-induced auto- and allo-specific cellular immune responses, play a major role in the loss of islets and islet function [14-16]. Persistence, or recurrence, of autoimmunity has been described in islet transplant recipients, and has been associated with lower rates of insulin independence and shorter graft survival [17]. Corroboratively, selective destruction of beta-cells within islet allografts, measurable changes of autoantibody levels (anti-GAD65 and anti-IA2), and/or detection of autoreactive cytotoxic and memory T cells to beta-cell-specific epitopes have been described [18]. Consequently, these data indicate that the detrimental impact of innate and adaptive immune responses is not fully contained by the Edmonton protocol-associated regimen of generalized immunosuppression. For this reason, alternative strategies aimed at selectively promoting islet survival, or inhibiting undesired islet-specific or non-specific immune responses, would be a step towards a better management and outcome of islet transplantation in diabetic patients, especially when viewed in terms of withdrawal of generalized immune suppression and long-lasting insulin independence [6, 19]. In this respect, stem cells could contribute to improved islet transplantation by 1. supporting the graft by increasing tissue revascularization and favoring beta-cell replication and/or survival, and 2. modulating immune response (Figure 1).
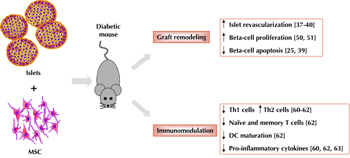 |
 |
Figure 1. Possible effects of islets plus mesenchymal stem cells co-transplantation on islet graft and immune system in diabetic murine models. |
|
Stem cells support islet graft by increasing tissue revascularization or favoring beta-cell replication and/or survival
Pancreatic islets are well vascularized throughout life. This is important for their ability to secrete insulin swiftly in response to changes in blood glucose. Although pancreatic islets comprise only 1-2% of pancreatic mass, they receive 5-10% of pancreatic blood flow. Islet isolation breaks the connections between the islet vasculature and the systemic circulation. Reestablishment of blood flow to transplanted islets requires several days. Rapid and adequate revascularization of transplanted islets is critical for islet survival and function. Endothelial cells are involved in an interdependent physical and functional relationship with beta-cells. They deliver oxygen and nutrients to endocrine cells, and provide a niche for beta-cells via the vascular basement membrane. In this regard, they help to induce insulin gene expression during islet development, sustain adult beta-cell function, promote beta-cell proliferation, and produce a number of vasoactive, angiogenic substances and growth factors [20, 21]. Delayed and insufficient revascularization can result in islet cell death and early graft failure. Therefore, strategies aimed at enhancing or accelerating this process are particularly needed.
Bone marrow- (BM) and tissue-derived adult stem cells are assumed to be involved in the process of islet revascularization. HSC, MSC, and endothelial progenitor cells (EPC) have all appeared as useful promoters of islet revascularization. Two predominant mechanisms have been identified, by which these cells contribute: 1. vessel formation by differentiation into mature endothelial cells (i.e. EPC) [22]. 2. Release of proangiogenic factors such as hepatocyte growth factor (HGF) and vascular endothelial growth factors A (VEGF A) [23, 24]. Mathews et al. provided evidence that transplanted BM-derived EPC are recruited to the pancreas in response to STZ-induced islet injury [25], and that EPC-mediated neovascularization of the pancreas can in principle facilitate the recovery of non-terminally injured beta-cells. Also, MSC have been shown to promote angiogenesis both in vivo [26] and in vitro [27]. However, it is not clear whether MSC have the potential to differentiate directly into endothelial cells, or whether they secrete angiogenic factors that stimulate EPC recruitment. Oswald et al. suggested that expanded adult human MSC can differentiate into cells with phenotypic and functional features of endothelial cells [28]. On the other hand, an increasing bulk of evidence supports the hypothesis that release of angiogenic factors rather than endothelial transdifferentiation is accountable for MSC-mediated angiogenesis [29, 30]. In this regard, Kinnaird et al. demonstrated that MSC contribute to tissue remodeling [31]. This is achieved by secreting a wide array of arteriogenic/angiogenic cytokines, including VEGF, fibroblast growth factor, angiopoetin-1, matrix metalloproteinases (MMP), and transforming growth factor-beta [32]. All these soluble mediators are able to support survival and differentiation of endothelial cells [33], and to promote neovascularization by mobilizing EPC [34].
Johansson and colleagues proposed an approach to promote islet revascularization based on the formation of a composite of endothelial cells, MSC, and islets [35]. This strategy provided evidence that the co-culture of MSC and endothelial cells with human islets in vitro before transplantation initiates formation of vessel-like structures that may promote islet engraftment after transplantation. Along the same line of argument, it has been confirmed in preclinical models that adult stem cell and islet co-localization in the same microenvironment after transplantation may improve tissue engraftment and survival.
In a syngeneic murine model of marginal islet mass transplantation, we reported that co-transplantation and co-localization of adult MSC with islets improve graft vascularization and function [36]. STZ-induced diabetic mice were transplanted under the kidney capsule with 200 syngeneic islets alternatively with, or without, 2.5 x 105 murine pancreas-derived MSC. The group receiving islets and MSC, showed a significantly better glycemic control, in terms of probability and time to reach euglycemia. The beneficial effect was not due to MSC differentiation into insulin-secreting cells. This was demonstrated by the fact that no insulin/GFP double positive cells were present when islets from wild-type mice, along with MSC derived from GFP+ transgenic mice, were co-transplanted. Instead, analysis of the graft revealed that CD31+ cell density was significantly higher in the presence of MSC, indicating that one of the benefits provided by MSC co-transplanta-tion is the increased neovascularization of the graft (Figure 2).
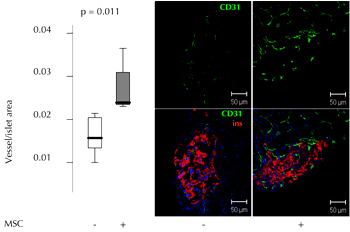 |
 |
Figure 2. Diabetic mice were transplanted with 200 autologous islets under the kidney capsule, alternatively with or without 2.5 x 105 pancreatic MSC. Left panel: quantitative evaluation of CD31+ cell area in islet graft with islets only (n = 7) or islets plus pMSC (n = 7) 3 weeks after transplantation. The vessel/islet area is shown for transplanted mice. Right panel: representative immunofluorescence images showing CD31 (green), insulin (red), and DAPI cell nuclei staining (blue) in islet graft with islets alone or islets plus pMSC (figure reproduced from [36]). |
|
Similarly, Figliuzzi et al. transplanted 2000 syngeneic islets alone, or in combination with 106 BM-derived MSC, under the kidney capsule of diabetic Lewis rats [37]. Animals transplanted with 2000 islets never reached normoglycemia. In contrast, rats transplanted with 2000 islets plus MSC, showed a gradual fall in glycemia after transplantation, with normoglycemia being maintained to the end of the experiment. Comparable glycemic control was obtained with transplantation of 3000 islets alone. The MSC preparation used for in vivo experiments expressed high levels of VEGF. Also revealed by this work, co-localization of MSC with islets significantly increased the number of capillaries, suggesting that the co-transplantation improved islet graft function and supported graft vascularization.
Two very recent papers further support the hypothesis that co-transplantation of islets and stem cells may be beneficial in terms of graft function and revascularization. Sakata et al. utilized total BM cells instead of MSC, and showed that the co-localization of MSC with islets is associated with enhanced graft function and vessel density [38]. In this study, STZ-induced diabetic mice were transplanted syngeneically under the kidney capsule with 100-200 islets alone, or in combination with 1-5 x 106 BM cells. Blood glucose was significantly lower in the islet/BM-cells group than in the islet-alone group after 63 days of transplantation. Also, significantly higher peri-islet vessel density and more prominent intra-islet VEGF staining were detected in the islet/BM-cells group than in the islet-alone group. There were no normoglycemic mice and no insulin-positive cells in the BM-cells-alone group, suggesting that a direct differentiation of BM cells in beta-cells was unlikely.
Ito et al. utilized liver instead of kidney capsule as site of co-transplantation. These authors found that co-transplantation of MSC and islets at this site promoted revascularization and improved islet graft function [39]. In the experiment, Lewis rat islets were infused into the liver of STZ-diabetic syngeneic recipients, with MSC isolated from Lewis rat BM. Co-trans-plantation of 500 islets and 107 MSC reversed diabetes in all eight recipients. Whereas, islet-alone transplantation achieved normoglycemia in only three of them. With 300 islets, diabetes was reversed in five of nine islet-MSC and one of ten islets-alone recipients. The results of intravenous glucose tolerance tests performed on day 56 were significantly better in islet-MSC than islet-alone recipients. One week after transplantation, well-pre-served islet structures and higher numbers of capillaries were found in the liver of islet-MSC recipients. In contrast, islet-alone grafts were fragmented and had few capillaries.
Mechanism of diabetes reversal through co-transplantation of islets and stem cells
It is reasonable to assume that the induced neovascularization plays a major role for proper islet engraftment and function. But the mechanism by which stem cells act as feeder tissue for islets in experiments remains unknown. This does not exclude the possibility that other mechanisms might also be involved. For example, in vitro studies reported that the MSC-derived laminin binding to α6β1-integrin expressed on pancreatic beta-cells could support insulin expression and beta-cell proliferation [20]. Similarly, MSC-derived collagen IV binding to α1β1-integrin could stimulate insulin secretion [40]. Moreover, the release from BM-derived cells of trophic molecules like HGF, IL-6, insulin-like growth factor binding proteins 4, VEGF A, and TGF-beta can directly sustain beta-cell survival and function [24, 41-44].
In vivo, a direct role has been proposed for stem cells in beta-cell replication, independent of the promotion of revascularization. Several recent studies have suggested that adult BM harbors cells able to influence beta-cell regeneration in diabetic animals [25, 43, 45-48]. For example, it has been shown that infusion of MSC in NOD/SCID mice, after STZ-induced islet destruction, can increase the number of endogenous beta-cells with a consecutive improvement of hyperglycemia [49]. These results have been corroborated by Urban and colleagues [50]. Freshly prepared sex-mismatched BM cells, and syngeneic or allogeneic MSC, were concomitantly administered into sub-lethally irradiated diabetic mice. After a single injection of a mixture of 106 BM cells per 105 MSC, blood glucose and serum insulin concentrations rapidly returned to normal levels, accompanied by efficient tissue regeneration. The authors suggested that two aspects of this successful treatment regimen could have operated in parallel, and synergistically, in the model. Firstly, BM cells and MSC induced the regeneration of recipient-derived pancreatic insulin-secreting cells. Secondly, MSC inhibited T-cell-mediated immune responses against newly formed beta-cells, which in turn were able to survive in this altered immunological milieu.
Recently, it has been proposed that non-mesodermal-derived stem cells support beta-cell replication. Different studies found that beta-cell growth may be affected by neurotrophins [51, 52], and that neural crest stem cells (NCSC) have an important role in beta-cell differentiation by regulating beta-cell mass during development [53]. On the basis of these observations, Olerud et al. investigated whether co-transplantation of islets and neurospheres derived from NCSC is beneficial for survival, growth, and function of transplanted beta-cells [54]. They co-transplanted NCSC with islets in diabetic mice. From the first days after transplantation NCSC developed interconnections with islet cells. Proliferation of beta-cells was markedly increased, and transplants displayed improved insulin release in mice receiving the mixed graft, compared with those receiving islet-alone transplants. This work is evidence for the hypothesis that stem cells develop direct contact with co-transplanted islets, and promote proliferation and growth of beta-cells. These effects are accompanied by a corresponding path of functional improvement.
Stem cells support islet graft by modulation of the immune response
One of the obstacles limiting clinical application of islet transplantation is the need for immune-suppressive therapies that are often associated with undesired side effects. Currently, this is the primary reason why the majority of type 1 diabetes patients cannot be offered islet transplantation. Thus, it is a major goal in islet transplantation to make immunosuppression obsolete.
In islet transplantation, stem cells could protect transplanted allogeneic islets by negatively regulating persistent T cell autoimmunity and by controlling the activation and effector function of alloreactive T cells. In addition, stem cell may suppress the activation and proliferation of B cells, and thereby impair the production of destructive auto- and allo-antibodies. Finally, they may prevent the differentiation and maturation of dendritic cells (DC). Thus, stem cells can prevent the destruction of transplanted islets that is usually caused by adaptive immune response. The immunomodulatory activity is a true functional signature reported for adult stem cells. For this activity, the most promising candidate (if not the only one) is the MSC. In fact, MSC immunosuppressive capabilities are well established. These cells might be used to control several subsets of immune cells, including naïve and memory T cells, B cells, DC, and NK cells, and to reduce inflammatory cytokine production [55]. In vivo animal transplant studies have shown MSC to be capable of preventing the rejection of skin and heart allografts in models [55-57]. Also, the use of MSC for the treatment of patients with graft-versus-host-disease after allogeneic BM transplantation has resulted in reliable therapeutic efficacy [58].
The first paper to address whether MSC co-transplantation allows for better islet graft acceptance with reduced requirement for immunosuppression was published in 2009 [59]. The ability of MSC to modulate the immune response was tested in a model of marginal mass islet transplantation. Allogeneic islet transplantation with or without syngeneic MSC was carried out in a rat model of STZ-induced diabetes by transplantation into the omental pouch. Islets plus MSC, but not islets alone, with short-term use of immunosuppression, enhanced long-term islet graft survival, improved insulin expression in the grafts, and induced normal serum insulin levels and normoglycemia. T cells from recipients transplanted with allogeneic islets plus MSC produced low levels of IFN-gamma and TNF-alpha upon ex-vivo activation. This transplantation protocol promoted the generation of IL-10-secreting CD4+ T cells.
The molecular mechanisms used by MSC in suppressing immune responses in allogeneic islet transplantation were then studied in more detail. It was demonstrated that MMP secreted by MSC play an important role in the suppressive activity of MSC. In particular, MMP-2 and MMP-9 reduced surface expression of CD25 on responding T cells. Blocking the activity of MMP-2 and MMP-9 in vitro completely abolished the suppression of T cell proliferation by MSC, and restored T cell expression of CD25 and responsiveness to interleukin-2 [60].
Recently, the suppressive effect of MSC on T lymphocyte subsets and DC was studied [61]. Allogeneic islets alone, or in combination with MSC, were transplanted under the kidney capsule of diabetic mice. Graft rejection was alleviated by the presence of MSC. Also, the ratios of T helper type 1 to T helper type 2 cell, and T cytotoxic type 1 to T cytotoxic type 2 cell, were reduced, and the numbers of naive and memory T cells were downregulated in peripheral blood after transplantation. Maturation, endocytosis, and interleukin-12 secretion of BM-derived DC from recipient mice were suppressed. The most recent study by Longoni and colleagues confirmed the ability of both syngeneic and allogeneic MSC to prevent acute rejection and improve glycemic control [62]. Transplantation of a marginal mass of pancreatic islets in rats with diabetes was accompanied by a triple-dose administration of MSC. Reduced glucose levels and low-grade rejections were observed up to 15 days after transplantation, indicating that MSC prolong graft function by preventing acute rejection. The efficacy of MSC was associated with a reduction of pro-inflammatory cytokines, and was independent of the administration route. Efficacy was similar for both syngeneic and allogeneic MSC, and comparable to immunosuppressive therapy.
In July 2010 the group of Norma Kenyon in Miami published a study about graft-promoting effects of MSC in a cynomolgus monkey model of islet/BM transplantation [63]. Allogeneic donor MSC were co-transplanted intraportally with islets on postoperative day (POD) 0, and intravenously with donor BM on POD 5 and 11. MSC treatment significantly enhanced islet engraftment and function at 1 month post-transplant, as compared to animals that received islets without MSC. Additional infusions of donor or third party MSC resulted in reversal of rejection episodes, and prolongation of islet function in 2 animals. Stable islet allograft function was associated with increased numbers of T regulatory cells in peripheral blood.
BM and BM-derived stem cells are easily available. Also, there is a wealth of experimental in-vitro and in-vivo data accompanied by widely consolidated clinical experience in the field of hematology. The combination of these aspects enabled the design of clinical trials involving adult stem cells to modulate the immune response in islet transplantation. The Miami group was the first to co-infuse pancreatic islets and stem cells in diabetic patients. It performed combined islet and donor CD34+ HSC infusion using an 'Edmonton-like' immunosuppression, without ablative conditioning [64]. The strategy was to induce recipient chimerism, and consequently graft tolerance. No graft-versus-host-disease, malignancy, or permanent complications were recorded. This outcome highlighted the relative safety of this protocol. But the co-transplantation did not lead to stable chimerism, and islet function was lost during follow up, or after immunosuppression withdrawal. At the moment, a new clinical trial is in progress in Miami. This trial again includes islet transplantation associated with CD34+-enriched donor BM cell infusion, but with a different immunosuppression protocol (ClinicalTrials.gov Identifier: NCT00315614). Meanwhile, another clinical trial is currently recruiting participants at Fuzhou General Hospital in China. This trial is aimed at evaluating safety and efficacy of islet/MSC co-transplantation in type 1 diabetic patients. The trial aims to assess the hypothesis that additional MSC infusion can support clinical islet transplantation by protection of islets from inflammatory damage, immunological modulation, and engraftment promotion (ClinicalTrials.gov identifier: NCT00646724).
It is also assumed that adult stem cells other than MSC have immunomodulatory properties when used in islet transplantation. We recently published the finding that the co-transplantation of NPC and pancreatic islets in a fully mismatched allograft model mediates tolerance [65]. Diabetic Balb/c mice were co-transplanted with pancreatic islets under the kidney capsule, and NPC from fully mismatched C57BL/6 mice. The co-transplantation and co-localization of NPC and islets induced stable long-term graft function in the absence of immunosuppression. This condition was associated with an expansion of CD4+CD25+FoxP3+ T regulatory cells in the spleen.
Safety concerns and identification of new sites for islet and stem cell co-localization: two open problems for clinical translation
Safety concerns
Some problems must be solved before stem cell/islet co-transplantation can be applied extensively in the clinic. As for stem cell therapies, One major challenge is safety. Adult stem cells have the advantage of a lower plasticity than embryonic or fetal stem cells. Also, their use as helper cells for supporting transplanted tissue is theoretically less risky than their use as differentiating progenitor cells, because they are not manipulated. Consequently, adult stem cells are less prone to genomic instability. On the other hand, as many as 5 to 200 x 106 stem cells/kg were used in animal studies [36-39]. Therefore, it is expected that in vitro culture and expansion of adult stem cells will be necessary to obtain sufficient numbers for use in clinical studies of co-transplantation. It will require the use of formulated liquid media supplemented with growth factors and other chemical substances to promote cellular replication. Cultured stem cells can accumulate genetic abnormalities as they replicate, and their rapid replication with any genetic instability can create a risk of tumor development. This risk was reported as high for murine stem cells. Several studies suggested that mouse MSC undergo spontaneous immortalization and malignant transformation after culture [66-69]. However, human stem cells appear to be more stable in culture, and the risk of tumor transformation appears to be low [66, 67, 70-72]. In two multi-center clinical trials, the immunologic and genetic features of human MSC, expanded with fetal calf serum and fibroblast growth factor, or with platelet lysate, were investigated in 4 cell-therapy facilities [72]. Cultured human MSC showed some recurring aneuploidy in vitro, independently of the culture process. However, MSC with or without chromosomal alterations showed progressive growth arrest, and entered senescence without evidence of transformation both in vitro and in vivo.
Despite the lower risk, there are well documented case reports of tumor development after stem cell injection in humans. One was a 9-year old boy with ataxia telangiectasia, who underwent fetal neural stem cell injection into the brain. Four years later, glioneural neoplasm has been diagnosed. Cellular and genetic analysis confirmed that the tumor arose from transplanted stem cells [73]. Another very recently reported case was a patient with lupus nephritis, treated by direct renal injection of autologous stem cells obtained from peripheral blood. The patient developed tissues at injection sites and hematuria, which were described as angiomyeloproliferative lesions, likely to have been induced by stem cells [74]. These cases, together with unreported experiences, led the International Society of Stem Cell Research to set up a professional guideline for clinics providing unproven stem cell based therapies [75]. This guideline requires a written plan for the procedure. It includes the scientific rationale, and any preclinical evidence of proof-of-principle for efficacy and safety, a full characterization of the cell types being transplanted, and the method of administration. Also recommended is an informed consent for patients, an action plan for adverse events, a timely move to a formal clinical trial after experience with a small number of patients, and the submission of systematic and objective tracking of outcomes to the scientific community for critical review.
It should be emphasized that, in the context of stem cell/islet co-transplantation, the genetic instability in culture before use is not a unique risk for tumor transformation. In our experience, co-transplantation of islets and NPC under the kidney capsule of diabetic mice was accompanied by constant and reproducible development of NPC-derived cancer [65]. However, we did not observe tumors in NPC transplanted alone, neither in allogeneic nor syngeneic settings. Therefore, we considered that cancer formation was mainly sustained by insulin secretion. This evidence introduces the concept that ‘safety’ of stem cell therapy, in terms of preventing cancer generation, is not an intrinsic stem cell feature, but also depends on cross talk with the microenvironment. In our model, insulin appeared to be responsible for the malignant transformation of NPC.
Sites for co-localization of islets and stem cells
The second major challenge for stem cell/islet co-transplantation in clinical practice involves the need to co-localize stem cells and islets. We are strongly convinced that co-infusion and co-localization of stem cells and target tissue in the same microenvironment is most important. The beneficial effect generated by stem cells very likely depends on cell-to-cell contact and release of key factors directly in the microenvironment. Some studies have compared in situ and systemic (intravenous) delivery of stem cells. They have reported a marked decrease in engraftment efficiency and function with intravenous delivery [76, 77]. Also, it was reported that intravenously infused MSC became trapped in the lung [78], where they appear as emboli in afferent blood vessels. It is possible that the small number of MSC that escaped being trapping in the lung, homed to the injured tissue and exerted a function. However, these findings suggest that the full potential of MSC can only be exploited by co-localization.
Co-transplantation and co-localization of stem cells and islets in humans is not feasible using intraliver islet infusion due to the spreading of tissue and cells in the vascular liver mass. Use of an extra-vascular site for islet engraftment is a possible solution to the problem. In theory, the BM offers an extra-vascular, protected, and well-vascularized (even if hypoxic) microenvironment, capable of sustaining islet grafts. Furthermore, BM is the natural environment of mesoderm-derived stem cells. Stem cells transplanted in BM may receive signals from this microenvironment that can favor their survival and function. In accordance with this hypothesis, we are currently exploring the possibility of using BM as the site for islet/stem cell co-transplantation and co-localization. In the past two years, we have observed that islet infusion in BM resulted in a significant improvement of transplantation outcome in mice. In fact, islets engrafted efficiently in the BM of diabetic mice. These animals showed a near-normal glucose metabolism for more than a year [79].
In a clinical trial at our institute, we have started to translate these results to five diabetic human patients. Thus far, it has shown that islets can survive and thrive in the BM [80]. This demonstrates that BM could be a feasible and alternative site for islet transplantation, and islet/stem cell co-transplantation, in humans. BM could be the microenvironment to support both function and survival of the two components as it permits physical co-localization.
Conclusions
In islet transplantation, co-infusion and co-localization with stem cells has been reported as an effective strategy to improve engraftment and prevent rejection. BM cells, and MSC in particular, seem to be the most promising candidates for islet/stem cell co-transplantation, due to their potential to sustain engraftment and modulate the immune response. Hopefully, this new therapy option will improve the possibility of obtaining long-term insulin independence, after transplantation of a reduced number of islets. This would facilitate broader application of islet transplantation.
Future studies in this field will have an impact on protocols involving stem cells. They will reveal the mechanisms through which stem cells exert their function as feeder and immunomodulatory cells.
Disclosures (conflict of interests statement): The authors report no conflict of interests.
Acknowledgments:
This work was supported by Fondazione Cariplo (2007-5165), EU FP7 (DIAPREPP, project no. 202013), and BetaCellTherapy - Beta Cell Therapy in Diabetes (project no. 241883).
References
- Martino G, Pluchino S. The therapeutic potential of neural stem cells. Nat Rev Neurosci 2006. 7(5):395-406. [DOD] [CrossRef]
- Franco OH, Steyerberg EW, Hu FB, Mackenbach J, Nusselder W. Associations of diabetes mellitus with total life expectancy and life expectancy with and without cardiovascular disease. Arch Intern Med 2007. 167(11):1145-1151. [DOD] [CrossRef]
- Hu FB, Stampfer MJ, Solomon CG, Liu S, Willett WC, Speizer FE, Nathan DM, Manson JE. The impact of diabetes mellitus on mortality from all causes and coronary heart disease in women: 20 years of follow-up. Arch Intern Med 2001. 161(14):1717-1723. [DOD] [CrossRef]
- Shapiro AM, Lakey JR, Ryan EA, Korbutt GS, Toth E, Warnock GL, Kneteman NM, Rajotte RV. Islet transplantation in seven patients with type 1 diabetes mellitus using a glucocorticoid-free immunosuppressive regimen. N Engl J Med 2000. 343(4):230-238. [DOD] [CrossRef]
- Froud T, Ricordi C, Baidal DA, Hafiz MM, Ponte G, Cure P, Pileggi A, Poggioli R, Ichii H, Khan A, et al. Islet transplantation in type 1 diabetes mellitus using cultured islets and steroid-free immunosuppression: Miami experience. Am J Transplant 2005. 5(8):2037-2046. [DOD] [CrossRef]
- Ricordi C. Islet transplantation: a brave new world. Diabetes 2003. 52(7):1595-1603. [DOD] [CrossRef]
- Vantyghem MC, Kerr-Conte J, Arnalsteen L, Sergent G, Defrance F, Gmyr V, Declerck N, Raverdy V, Vandewalle B, Pigny P, Noel C, Pattou F. Primary graft function, metabolic control, and graft survival after islet transplantation. Diabetes Care 2009. 32(8):1473-1478. [DOD] [CrossRef]
- Shapiro AM, Ricordi C, Hering BJ, Auchincloss H, Lindblad R, Robertson RP, Secchi A, Brendel MD, Berney T, Brennan DC, et al. International trial of the Edmonton protocol for islet transplantation. N Engl J Med 2006. 355(13):1318-1330. [DOD] [CrossRef]
- Cure P, Pileggi A, Froud T, Messinger S, Faradji RN, Baidal DA, Cardani R, Curry A, Poggioli R, Pugliese A, et al. Improved metabolic control and quality of life in seven patients with type 1 diabetes following islet after kidney transplantation. Transplantation 2008. 85(6):801-812. [DOD] [CrossRef]
- Hering BJ, Kandaswamy R, Ansite JD, Eckman PM, Nakano M, Sawada T, Matsumoto I, Ihm SH, Zhang HJ, Parkey J, Hunter DW, Sutherland DE. Single-donor, marginal-dose islet transplantation in patients with type 1 diabetes. JAMA 2005. 293(7):830-835. [DOD] [CrossRef]
- Tan J, Yang S, Cai J, Guo J, Huang L, Wu Z, Chen J, Liao L. Simultaneous islet and kidney transplantation in seven patients with type 1 diabetes and end-stage renal disease using a glucocorticoid-free immunosuppressive regimen with alemtuzumab induction. Diabetes 2008. 57(10):2666-2671. [DOD] [CrossRef]
- Ryan EA, Paty BW, Senior PA, Bigam D, Alfadhli E, Kneteman NM, Lakey JR, Shapiro AM. Five-year follow-up after clinical islet transplantation. Diabetes 2005. 54(7):2060-2069. [DOD] [CrossRef]
- Ryan EA, Lakey JR, Paty BW, Imes S, Korbutt GS, Kneteman NM, Bigam D, Rajotte RV, Shapiro AM. Successful islet transplantation: continued insulin reserve provides long-term glycemic control. Diabetes 2002. 51(7):2148-2157. [DOD] [CrossRef]
- Mohanakumar T, Narayanan K, Desai N, Ramachandran S, Shenoy S, Jendrisak M, Susskind BM, Olack B, Benshoff N, Phelan DL, et al. A significant role for histocompatibility in human islet transplantation. Transplantation 2006. 82(2):180-187. [DOD] [CrossRef]
- Cardani R, Pileggi A, Ricordi C, Gomez C, Baidal DA, Ponte GG, Mineo D, Faradji RN, Froud T, Ciancio G, et al. Allosensitization of islet allograft recipients. Transplantation 2007. 84(11):1413-1427. [DOD] [CrossRef]
- Campbell PM, Senior PA, Salam A, Labranche K, Bigam DL, Kneteman NM, Imes S, Halpin A, Ryan EA, Shapiro AM. High risk of sensitization after failed islet transplantation. Am J Transplant 2007. 7(10):2311-2317. [DOD] [CrossRef]
- Bosi E, Braghi S, Maffi P, Scirpoli M, Bertuzzi F, Pozza G, Secchi A, Bonifacio E. Autoantibody response to islet transplantation in type 1 diabetes. Diabetes 2001. 50(11):2464-2471. [DOD] [CrossRef]
- Huurman VA, Hilbrands R, Pinkse GG, Gillard P, Duinkerken G, van de Linde P, van der Meer-Prins PM, Versteeg-van der Voort Maarschalk MF, Verbeeck K, Alizadeh BZ, et al. Cellular islet autoimmunity associates with clinical outcome of islet cell transplantation. PLoS One 2008. 3(6):e2435. [DOD] [CrossRef]
- Pileggi A, Cobianchi L, Inverardi L, Ricordi C. Overcoming the challenges now limiting islet transplantation: a sequential, integrated approach. Ann N Y Acad Sci 2006. 1079:383-398. [DOD] [CrossRef]
- Nikolova G, Strilic B, Lammert E. The vascular niche and its basement membrane. Trends Cell Biol 2007. 17(1):19-25. [DOD] [CrossRef]
- Zanone MM, Favaro E, Camussi G. From endothelial to beta cells: insights into pancreatic islet microendothelium. Curr Diabetes Rev 2008. 4(1):1-9. [DOD] [CrossRef]
- Asahara T, Murohara T, Sullivan A, Silver M, van der Zee R, Li T, Witzenbichler B, Schatteman G, Isner JM. Isolation of putative progenitor endothelial cells for angiogenesis. Science 1997. 275(5302):964-967. [DOD] [CrossRef]
- Di Santo S, Yang Z, Wyler von Ballmoos M, Voelzmann J, Diehm N, Baumgartner I, Kalka C. Novel cell-free strategy for therapeutic angiogenesis: in vitro generated conditioned medium can replace progenitor cell transplantation. PLoS One 2009. 4(5):e5643. [DOD] [CrossRef]
- Park KS, Kim YS, Kim JH, Choi B, Kim SH, Tan AH, Lee MS, Lee MK, Kwon CH, Joh JW, Kim SJ, Kim KW. Trophic molecules derived from human mesenchymal stem cells enhance survival, function, and angiogenesis of isolated islets after transplantation. Transplantation 2010. 89(5):509-517. [DOD]
- Mathews V, Hanson PT, Ford E, Fujita J, Polonsky KS, Graubert TA. Recruitment of bone marrow-derived endothelial cells to sites of pancreatic beta-cell injury. Diabetes 2004. 53(1):91-98. [DOD] [CrossRef]
- Al-Khaldi A, Eliopoulos N, Martineau D, Lejeune L, Lachapelle K, Galipeau J. Postnatal bone marrow stromal cells elicit a potent VEGF-dependent neoangiogenic response in vivo. Gene Ther 2003. 10(8):621-629. [DOD] [CrossRef]
- Gruber R, Kandler B, Holzmann P, Vögele-Kadletz M, Losert U, Fischer MB, Watzek G. Bone marrow stromal cells can provide a local environment that favors migration and formation of tubular structures of endothelial cells. Tissue Eng 2005. 11(5-6):896-903. [DOD] [CrossRef]
- Oswald J, Boxberger S, Jorgensen B, Feldmann S, Ehninger G, Bornhäuser M, Werner C. Mesenchymal stem cells can be differentiated into endothelial cells in vitro. Stem Cells 2004. 22(3):377-384. [DOD] [CrossRef]
- Javazon EH, Keswani SG, Badillo AT, Crombleholme TM, Zoltick PW, Radu AP, Kozin ED, Beggs K, Malik AA, Flake AW. Enhanced epithelial gap closure and increased angiogenesis in wounds of diabetic mice treated with adult murine bone marrow stromal progenitor cells. Wound Repair Regen 2007. 15(3):350-359. [DOD] [CrossRef]
- Wu Y, Chen L, Scott PG, Tredget EE. Mesenchymal stem cells enhance wound healing through differentiation and angiogenesis. Stem Cells 2007. 25(10):2648-2659. [DOD] [CrossRef]
- Kinnaird T, Stabile E, Burnett MS, Shou M, Lee CW, Barr S, Fuchs S, Epstein SE. Local delivery of marrow-derived stromal cells augments collateral perfusion through paracrine mechanisms. Circulation 2004. 109(12):1543-1549. [DOD] [CrossRef]
- Kinnaird T, Stabile E, Burnett MS, Lee CW, Barr S, Fuchs S, Epstein SE. Marrow-derived stromal cells express genes encoding a broad spectrum of arteriogenic cytokines and promote in vitro and in vivo arteriogenesis through paracrine mechanisms. Circ Res 2004. 94(5):678-685. [DOD] [CrossRef]
- Kaigler D, Krebsbach PH, Polverini PJ, Mooney DJ. Role of vascular endothelial growth factor in bone marrow stromal cell modulation of endothelial cells. Tissue Eng 2003. 9(1):95-103. [DOD] [CrossRef]
- Asahara T, Takahashi T, Masuda H, Kalka C, Chen D, Iwaguro H, Inai Y, Silver M, Isner JM. VEGF contributes to postnatal neovascularization by mobilizing bone marrow-derived endothelial progenitor cells. EMBO J 1999. 18(14):3964-3972. [DOD] [CrossRef]
- Johansson U, Rasmusson I, Niclou SP, Forslund N, Gustavsson L, Nilsson B, Korsgren O, Magnusson PU. Formation of composite endothelial cell-mesenchymal stem cell islets: a novel approach to promote islet revascularization. Diabetes 2008. 57(9):2393-2401. [DOD] [CrossRef]
- Sordi V, Melzi R, Mercalli A, Formicola R, Doglioni C, Tiboni F, Ferrari G, Nano R, Chwalek K, Lammert E, et al. Mesenchymal cells appearing in pancreatic tissue culture are bone marrow-derived stem cells with the capacity to improve transplanted islet function. Stem Cells 2010. 28(1):140-151. [DOD]
- Figliuzzi M, Cornolti R, Perico N, Rota C, Morigi M, Remuzzi G, Remuzzi A, Benigni A. Bone marrow-derived mesenchymal stem cells improve islet graft function in diabetic rats. Transplant Proc 2009. 41(5):1797-1800. [DOD] [CrossRef]
- Sakata N, Chan NK, Chrisler J, Obenaus A, Hathout E. Bone marrow cell cotransplantation with islets improves their vascularization and function. Transplantation 2010. 89(6):686-693. [DOD] [CrossRef]
- Ito T, Itakura S, Todorov I, Rawson J, Asari S, Shintaku J, Nair I, Ferreri K, Kandeel F, Mullen Y. Mesenchymal stem cell and islet co-transplantation promotes graft revascularization and function. Transplantation 2010. 89(12):1438-1445. [DOD] [CrossRef]
- Kaido T, Yebra M, Cirulli V, Montgomery AM. Regulation of human beta-cell adhesion, motility, and insulin secretion by collagen IV and its receptor alpha1beta1. J Biol Chem 2004. 279(51):53762-53769. [DOD] [CrossRef]
- Chao KC, Chao KF, Chen CF, Liu SH. A novel human stem cell coculture system that maintains the survival and function of culture islet-like cell clusters. Cell Transplant 2008. 17(6):657-664. [DOD] [CrossRef]
- Choi SE, Choi KM, Yoon IH, Shin JY, Kim JS, Park WY, Han DJ, Kim SC, Ahn C, Kim JY, et al. IL-6 protects pancreatic islet beta cells from pro-inflammatory cytokines-induced cell death and functional impairment in vitro and in vivo. Transpl Immunol 2004. 13(1):43-53. [DOD] [CrossRef]
- Izumida Y, Aoki T, Yasuda D, Koizumi T, Suganuma C, Saito K, Murai N, Shimizu Y, Hayashi K, Odaira M, et al. Hepatocyte growth factor is constitutively produced by donor-derived bone marrow cells and promotes regeneration of pancreatic beta-cells. Biochem Biophys Res Commun 2005. 333(1):273-282. [DOD] [CrossRef]
- Jabs N, Franklin I, Brenner MB, Gromada J, Ferrara N, Wollheim CB, Lammert E. Reduced insulin secretion and content in VEGF-a deficient mouse pancreatic islets. Exp Clin Endocrinol Diabetes 2008. 116(Suppl 1):S46-S49. [DOD] [CrossRef]
- Wang X, Willenbring H, Akkari Y, Torimaru Y, Foster M, Al-Dhalimy M, Lagasse E, Finegold M, Olson S, Grompe M. Cell fusion is the principal source of bone-marrow-derived hepatocytes. Nature 2003. 422(6934):897-901. [DOD] [CrossRef]
- Luo L, Badiavas E, Luo JZ, Maizel A. Allogeneic bone marrow supports human islet beta cell survival and function over six months. Biochem Biophys Res Commun 2007. 361(4):859-864. [DOD] [CrossRef]
- Hasegawa Y, Ogihara T, Yamada T, Ishigaki Y, Imai J, Uno K, Gao J, Kaneko K, Ishihara H, Sasano H, et al. Bone marrow (BM) transplantation promotes beta-cell regeneration after acute injury through BM cell mobilization. Endocrinology 2007. 148(5):2006-2015. [DOD] [CrossRef]
- Li FX, Zhu JW, Tessem JS, Beilke J, Varella-Garcia M, Jensen J, Hogan CJ, DeGregori J. The development of diabetes in E2f1/E2f2 mutant mice reveals important roles for bone marrow-derived cells in preventing islet cell loss. Proc Natl Acad Sci USA 2003. 100(22):12935-12940. [DOD] [CrossRef]
- Lee RH, Seo MJ, Reger RL, Spees JL, Pulin AA, Olson SD, Prockop DJ. Multipotent stromal cells from human marrow home to and promote repair of pancreatic islets and renal glomeruli in diabetic NOD/scid mice. Proc Natl Acad Sci USA 2006. 103(46):17438-17443. [DOD] [CrossRef]
- Urban VS, Kiss J, Kovacs J, Gocza E, Vas V, Monostori E, Uher F. Mesenchymal stem cells cooperate with bone marrow cells in therapy of diabetes. Stem Cells 2008. 26(1):244-253. [DOD] [CrossRef]
- Miao G, Mace J, Kirby M, Hopper A, Peverini R, Chinnock R, Shapiro J, Hathout E. In vitro and in vivo improvement of islet survival following treatment with nerve growth factor. Transplantation 2006. 81(4):519-524. [DOD] [CrossRef]
- Teitelman G, Guz Y, Ivkovic S, Ehrlich M. Islet injury induces neurotrophin expression in pancreatic cells and reactive gliosis of peri-islet Schwann cells. J Neurobiol 1998. 34(4):304-318. [DOD] [CrossRef]
- Nekrep N, Wang J, Miyatsuka T, German MS. Signals from the neural crest regulate beta-cell mass in the pancreas. Development 2008. 135(12):2151-2160. [DOD] [CrossRef]
- Olerud J, Kanaykina N, Vasylovska S, King D, Sandberg M, Jansson L, Kozlova EN. Neural crest stem cells increase beta cell proliferation and improve islet function in co-transplanted murine pancreatic islets. Diabetologia 2009. 52(12):2594-2601. [DOD] [CrossRef]
- Nauta AJ, Fibbe WE. Immunomodulatory properties of mesenchymal stromal cells. Blood 2007. 110(10):3499-3506. [DOD] [CrossRef]
- Bartholomew A, Sturgeon C, Siatskas M, Ferrer K, McIntosh K, Patil S, Hardy W, Devine S, Ucker D, Deans R, Moseley A, Hoffman R. Mesenchymal stem cells suppress lymphocyte proliferation in vitro and prolong skin graft survival in vivo. Exp Hematol 2002. 30(1):42-48. [DOD] [CrossRef]
- Casiraghi F, Azzollini N, Cassis P, Imberti B, Morigi M, Cugini D, Cavinato RA, Todeschini M, Solini S, Sonzogni A, Perico N, Remuzzi G, Noris M. Pretransplant infusion of mesenchymal stem cells prolongs the survival of a semiallogeneic heart transplant through the generation of regulatory T cells. J Immunol 2008. 181(6):3933-3946. [DOD]
- von Bonin M, Stölzel F, Goedecke A, Richter K, Wuschek N, Hölig K, Platzbecker U, Illmer T, Schaich M, Schetelig J, et al. Treatment of refractory acute GVHD with third-party MSC expanded in platelet lysate-containing medium. Bone Marrow Transplant 2009. 43(3):245-251. [DOD] [CrossRef]
- Solari MG, Srinivasan S, Boumaza I, Unadkat J, Harb G, Garcia-Ocana A, Feili-Hariri M. Marginal mass islet transplantation with autologous mesenchymal stem cells promotes long-term islet allograft survival and sustained normoglycemia. J Autoimmun 2009. 32(2):116-124. [DOD] [CrossRef]
- Ding Y, Xu D, Feng G, Bushell A, Muschel RJ, Wood KJ. Mesenchymal stem cells prevent the rejection of fully allogenic islet grafts by the immunosuppressive activity of matrix metalloproteinase-2 and -9. Diabetes 2009. 58(8):1797-1806. [DOD] [CrossRef]
- Li FR, Wang XG, Deng CY, Qi H, Ren LL, Zhou HX. Immune modulation of co-transplantation mesenchymal stem cells with islet on T and dendritic cells. Clin Exp Immunol 2010. 161(2):357-363. [DOD]
- Longoni B, Szilagyi E, Quaranta P, Paoli GT, Tripodi S, Urbani S, Mazzanti B, Rossi B, Fanci R, Demontis GC, et al. Mesenchymal stem cells prevent acute rejection and prolong graft function in pancreatic islet transplantation. Diabetes Technol Ther 2010. 12(6):435-446. [DOD] [CrossRef]
- Berman DM, Willman MA, Han D, Kleiner G, Kenyon NM, Cabrera O, Karl JA, Wiseman RW, O’Connor DH, Bartholomew AM, Kenyon NS. Mesenchymal stem cells enhance allogeneic islet engraftment in nonhuman primates. Diabetes 2010. In press. [DOD]
- Mineo D, Ricordi C, Xu X, Pileggi A, Garcia-Morales R, Khan A, Baidal DA, Han D, Monroy K, Miller J, et al. Combined islet and hematopoietic stem cell allotransplantation: a clinical pilot trial to induce chimerism and graft tolerance. Am J Transplant 2008. 8(6):1262-1274. [DOD] [CrossRef]
- Melzi R, Antonioli B, Mercalli A, Battaglia M, Valle A, Pluchino S, Galli R, Sordi V, Bosi E, Martino G, et al. Co-graft of allogeneic immune regulatory neural stem cells (NPC) and pancreatic islets mediates tolerance, while inducing NPC-derived tumors in mice. PLoS One 2010. 5(4):e10357. [DOD] [CrossRef]
- Aguilar S, Nye E, Chan J, Loebinger M, Spencer-Dene B, Fisk N, Stamp G, Bonnet D, Janes SM. Murine but not human mesenchymal stem cells generate osteosarcoma-like lesions in the lung. Stem Cells 2007. 25(6):1586-1594. [DOD] [CrossRef]
- Miura M, Miura Y, Padilla-Nash HM, Molinolo AA, Fu B, Patel V, Seo BM, Sonoyama W, Zheng JJ, Baker CC, Chen W, Ried T, Shi S. Accumulated chromosomal instability in murine bone marrow mesenchymal stem cells leads to malignant transformation. Stem Cells 2006. 24(4):1095-1103. [DOD] [CrossRef]
- Bernardo ME, Zaffaroni N, Novara F, Cometa AM, Avanzini MA, Moretta A, Montagna D, Maccario R, Villa R, Daidone MG, Zuffardi O, Locatelli F. Human bone marrow derived mesenchymal stem cells do not undergo transformation after long-term in vitro culture and do not exhibit telomere maintenance mechanisms. Cancer Res 2007. 67(19):9142-9149. [DOD] [CrossRef]
- Zhang ZX, Guan LX, Zhang K, Wang S, Cao PC, Wang YH, Wang Z, Dai LJ. Cytogenetic analysis of human bone marrow-derived mesenchymal stem cells passaged in vitro. Cell Biol Int 2007. 31(6):645-648. [DOD] [CrossRef]
- Zhou YF, Bosch-Marce M, Okuyama H, Krishnamachary B, Kimura H, Zhang L, Huso DL, Semenza GL. Spontaneous transformation of cultured mouse bone marrow-derived stromal cells. Cancer Res 2006. 66(22):10849-10854. [DOD] [CrossRef]
- Tolar J, Nauta AJ, Osborn MJ, Panoskaltsis Mortari A, McElmurry RT, Bell S, Xia L, Zhou N, Riddle M, Schroeder TM, et al. Sarcoma derived from cultured mesenchymal stem cells. Stem Cells 2007. 25(2):371-379. [DOD] [CrossRef]
- Tarte K, Gaillard J, Lataillade JJ, Fouillard L, Becker M, Mossafa H, Tchirkov A, Rouard H, Henry C, Splingard M, et al. Clinical-grade production of human mesenchymal stromal cells: occurrence of aneuploidy without transformation. Blood 2010. 115(8):1549-1553. [DOD] [CrossRef]
- Amariglio N, Hirshberg A, Scheithauer BW, Cohen Y, Loewenthal R, Trakhtenbrot L, Paz N, Koren-Michowitz M, Waldman D, Leider-Trejo L, Toren A, Constantini S, Rechavi G. Donor-derived brain tumor following neural stem cell transplantation in an ataxia telangiectasia patient. PLoS Med 2009. 6(2):e1000029. [DOD]
- Thirabanjasak D, Tantiwongse K, Thorner PS. Angiomyeloproliferative lesions following autologous stem cell therapy. J Am Soc Nephrol 2010. 21(7):1218-1222. [DOD] [CrossRef]
- ISSCR Guidelines for the Clinical Translation of Stem Cells. Curr Protoc Stem Cell Biol 2009. Appendix 1:Appendix 1B. [DOD]
- de Haro J, Zurita M, Ayllon L, Vaquero J. Detection of 111In-oxine-labeled bone marrow stromal cells after intravenous or intralesional administration in chronic paraplegic rats. Neurosci Lett 2005. 377(1):7-11. [DOD] [CrossRef]
- Paul C, Samdani AF, Betz RR, Fischer I, Neuhuber B. Grafting of human bone marrow stromal cells into spinal cord injury: a comparison of delivery methods. Spine (Phila Pa 1976) 2009. 34(4):328-334. [DOD]
- Gao J, Dennis JE, Muzic RF, Lundberg M, Caplan AI. The dynamic in vivo distribution of bone marrow-derived mesenchymal stem cells after infusion. Cells Tissues Organs 2001. 169(1):12-20. [DOD] [CrossRef]
- Cantarelli E, Melzi R, Mercalli A, Sordi V, Ferrari G, Lederer CW, Mrak E, Rubinacci A, Ponzoni M, Sitia G, Guidotti LG, Bonifacio E, Piemonti L. Bone marrow as an alternative site for islet transplantation. Blood 2009. 114(20):4566-4574. [DOD] [CrossRef]
- Piemonti L. Engraftment of pancreatic islets in bone marrow: preclinical evaluation and pilot clinical experience. 36th Annual Meeting of the European Group for Blood and Marrow Transplantation. Vienna, Austria, March 21-24, 2010. Oral presentation. [DOD]
This article has been cited by other articles:
|
IL-12 involvement in myogenic differentiation of C2C12 in vitro
Romanazzo S, Forte G, Morishimace K, Taniguchi A
Biomaterial Sci 2015. 2015(3):469-479
|
|
|
Bone marrow- and cord blood-derived stem cell transplantation for diabetes therapy
Cantarelli E, Pellegrini S, Citro A, Sordi V, Piemonti L
CellR4 2015. 3(1):e1408
|
|
|
Manipulation of cell sorting within mesenchymal stromal cell-islet cell multicellular spheroids
Hoffecker IT, Iwata H
Tissue Eng Part A 2014. 20(11-12):1643-1653
|
|
|
Design of bioartificial pancreas with functional micro/nano-based encapsulation of islets
Kepsutlu B, Nazli C, Bal T, Kizilel S
Curr Pharm Biotechnol 2014. 15(7):590-608
|
|
|
Selected cytokines in patients with pancreatic cancer: a preliminary report
Błogowski W, Deskur A, Budkowska M, Sałata D, Madej-Michniewicz A, Dabkowski K, Dolegowska B, Starzynska T
Plos One 2014. 9(5):e97613
|
|
|
In vivo bioluminescence imaging of transplanted mesenchymal stem cells as a potential source for pancreatic regeneration
Lee S, Youn H, Chung T, Hwang do W, Oh SW, Kang KW, Chung JK, Lee DS
Mol Imaging 2014. 2014:13
|
|
|
The impact of oxidative stress on islet transplantation and monitoring the graft survival by non-invasive imaging
Ramkumar KM, Sekar TV, Bhakkiyalakshmi E, Foygel K, Rajaguru P, Berger F, Paulmurugan R
Curr Med Chem 2013. 20(9):1127-1146
|
|
|
Contribution of murine bone marrow mesenchymal stem cells to pancreas regeneration after partial pancreatectomy in mice
Han F, Wang CY, Yang L, Zhan SD, Zhang M, Tian K
Cell Biol Int 2012. 36(9):823-831
|
|
|
A Step-up Approach for Cell Therapy in Stroke: Translational Hurdles of Bone Marrow-Derived Stem Cells
Glover LE, Tajiri N, Weinbren NL, Ishikawa H, Shinozuka K, Kaneko Y, Watterson DM, Borlongan CV
Transl Stroke Res 2012. 3(1):90-98
|
|
|
Genetically engineered islets and alternative sources of insulin-producing cells for treating autoimmune diabetes: quo vadis?
Chou FC, Huang SH, Sytwu HK
Int J Endocrinol 2012. 2012:296485
|
|
|
The great migration of bone marrow-derived stem cells toward the ischemic brain: therapeutic implications for stroke and other neurological disorders
Borlongan CV, Glover LE, Tajiri N, Kaneko Y, Freeman TB
Prog Neurobiol 2011. 95(2):213-228
|
|
|
New hope for type 2 diabetics: targeting insulin resistance through the immune modulation of stem cells
Zhao Y, Jiang Z, Guo C
Autoimmun Rev 2011. 11(2):137-142
|
|
|
A step-up approach for cell therapy in stroke: translational hurdles of bone marrow-derived stem cells
Glover LE, Tajiri N, Weinbren NL, Ishikawa H, Shinozuka K, Kaneko Y, Watterson DM, Borlongan CV
Transl Stroke Res 2011. In press
|
|
|
Current status of immunomodulatory and cellular therapies in preclinical and clinical islet transplantation
Chhabra P, Brayman KL
J Transplant 2011. 2011:637692
|
|
|
Beta-cell generation: can rodent studies be translated to humans?
Carlotti F, Zaldumbide A, Ellenbroek JH, Spijker HS, Hoeben RC, de Koning EJ
J Transplant 2011. 2011:892453
|
|
|
Human placenta-derived mesenchymal stem cells and islet-like cell clusters generated from these cells as a novel source for stem cell therapy in diabetes
Kadam S, Muthyala S, Nair P, Bhonde R
Rev Diabet Stud 2010. 7(2):168-182
|
|
|