Review
Rev Diabet Stud,
2011,
8(3):382-391 |
DOI 10.1900/RDS.2011.8.382 |
Cardiovascular Effects of Incretin-Based Therapies
Michael Lehrke, Nikolaus Marx
Department of Internal Medicine I, University Hospital Aachen, Pauwelsstraße 30, 52074 Aachen, Germany
Address correspondence to: Michael Lehrke, e-mail: mlehrke@ukaachen.de
Manuscript submitted July 7, 2011; resubmitted July 17, 2011; accepted July 22, 2011.
Keywords: type 2 diabetes, hypoglycemia, glycemic control, antidiabetic drug, incretin, GLP-1, DPP-4, exendin-4, liraglutide, cardioprotective
Abstract
GLP-1-modulating therapies are a class of anti-diabetic drugs that improve glycemic control by stimulating glucose-dependent insulin secretion from pancreatic beta-cells. In addition, GLP-1-based therapies have a variety of extrapancreatic effects, including satiety induction and gastric mobility reduction, which extend to distinct cardiovascular actions. GLP-1 was found to reduce infarct size in the context of acute myocardial ischemia which depends on the activation of prosurvival pathways including PI3-kinase, Akt, and ERK1/2. Also, GLP-1 augments the left ventricular function in dilative and metabolic cardiomyopathy, possibly by increasing insulin independent cardiomyocyte glucose uptake. Furthermore, experimental and preliminary clinical evidence suggest vasoprotective efficacy of GLP-1 mediated by improved endothelial function and anti-inflammatory capacities leading to atheroprotection. Mechanistically, the GLP-1 receptor is relevant for glucose lowering efficacy of GLP-1. However, many of its vasoprotective actions have also been described for the GLP-1 metabolite (9-37), which does not activate the GLP-1 receptor, suggesting the presence of an additional, yet unknown, signaling pathway. Ongoing research investigates the relevance of these observations in human disease and underlying mechanisms, which are reviewed in the present article.
Abbreviations: AGE - advanced glycation end product; AMPK - AMP-activated protein kinase; ApoE - apolipoprotein E; BAD - Bcl-2-associated death promoter; cAMP - cyclic adenosine monophosphate; CVD - cardiovascular disease; DPP-4 - dipeptidyl peptidase-4; eNOS - endothelial nitric oxide synthase; ERK1/2 - extracellular signal-regulated kinases 1/2; GLP-1 - glucagon-like peptide-1; GLUT4 - glucose transporter type 4; GSK - glycogen synthase kinase; HbA1c - glycated hemoglobin; HDL - high-density lipoprotein; LPS - lipopolysaccharide; MAP - mitogen-activated protein; MAPK - mitogen-activated protein kinase; mTOR - mammalian target of rapamycin; NF-κB - nuclear factor-kappa B; NOS2 - nitric oxide synthase 2; Nrf2 - nuclear factor (erythroid-derived 2)-like 2; NYHA - New York Heart Association; PC1/3 - prohormone convertase 1/3; PI3K - phosphoinositol 3-kinase; PKA - protein kinase A; PPAR - peroxisome proliferator-activated receptor; RAGE - receptor for advanced glycation end-products; RISK - reperfusion injury salvage kinase; UCP3 - uncoupling protein 3; UKPDS - United Kingdom Prospective Diabetes Study
Introduction
An epidemic growth of obesity parallels the world wide expansion of the western life style. This is associated with a dramatic increase of insulin resistance and type 2 diabetes as primary risk factors for cardiovascular disease and heart failure.
While an inverse association between glycemic control and mortality is well established, data demonstrating a prognostic benefit of glucose-lowering therapies in diabetic patients are limited. Although intensive blood glucose control reduces microvascular complications, effects on macrovascular disease, or mortality, remain disappointing [1]. Interestingly, improved prognostic outcome has been reported for some antidiabetic drugs, but not for others despite similar HbA1c-lowering capacities. Metformin was found to reduce mortality in a subpopulation of the United Kingdom Prospective Diabetes Study (UKPDS) and in other retrospective cohort studies [2-5]. This suggests strong relevance of drug-specific, HbA1c-independent effects for overall prognosis. In case of metformin, this might be due to its favorable side effects, namely body weight reduction and avoidance of hypoglycemia [6]. Therefore, the evaluation of new antidiabetic drugs should include a thorough metabolic and cardiovascular risk assessment.
GLP-1-modulating drugs have been introduced to the clinics in recent years. High expectations for favorable prognostic effects of these drugs have been raised by their potent HbA1c-lowering capacity, and their beneficial effect on body weight and the avoidance of hypoglycemia. These are potential key elements for cardioprotective therapy.
GLP-1 is an incretin hormone which is secreted by the intestinal L-cells in response to nutritional stimuli [7]. Circulatory GLP-1 binds to the GLP-1 receptor expressed on pancreatic beta-cell, leading to increased glucose-dependent insulin secretion. In addition, GLP-1 inhibits glucagon release from pancreatic alpha-cells and impairs gastric emptying, which in concert improves postprandial glucose metabolism [8]. Finally, GLP-1 has beta-cell protective, antiapoptotic effects, and induces satiety by stimulation central hypothalamic neurons [7, 8].
GLP-1 is produced in a protein convertase PC1/3-dependent manner from the preproglucagon gene which also harbors glucagon, GLP-2, and oxyntomodulin [9]. This leads to the secretion of GLP-1 (7-36-amide) and GLP-1 (7-37) which both feature similar activity on the GLP-1 receptor [10]. The half life of GLP-1 is limited to approximately 2 minutes. The ubiquitously present enzyme dipeptidyl peptidase-4 (DPP-4) cleaves off the first two amino acids of the peptide leading to rapid inactivation [7]. Thereby, the N-terminal-truncated GLP-1 metabolite (9-36-amide), or (9-37), is created which is unable to activate the GLP-1 receptor and rather act as a weak receptor antagonist [11-13]. Consistently, in vivo application of the GLP-1 metabolite (9-36) does not increase insulin secretion in pigs or humans [14, 15]. However, a number of reports suggest a cardiovascular effect of the GLP-1 metabolite [16].
Signal transduction of GLP-1 is mediated via the GLP-1 receptor which belongs to the glucagon superfamily of G-protein-coupled receptors and functions by increasing intracellular cyclic adenosine monophosphate (cAMP), calcium, and phospholipase C [7, 17]. Expression of the GLP-1 receptor is found in a variety of tissues including pancreatic islet cells, lung, heart, kidney, stomach, brain, endothelial cells, vascular smooth muscle cells, and cardiomyocytes [7].
The improvement of glucose metabolism by the multifactorial actions of GLP-1 has let to the development of a variety of new drugs which either mimic the GLP-1 peptide or increase the bioactivity of active, endogenous GLP-1 by inhibition of its degrading enzyme DPP-4 [17]. So far, two GLP-1 agonists have been approved for the clinic. These are exendin-4 and liraglutide [17]. Exendin-4 is a natural DPP-4-resistant peptide with only 53% homology to GLP-1 but full receptor activating capacity. Liraglutide, however, is a fatty acid-modified GLP-1 homolog which displays a prolonged half life by increased albumin binding capacity. Both agonists similarly improve glucose metabolism and cause HbA1c reduction by approximately 1%. Another positive effect is that they promote weight loss and thereby favorably impact on the vicious cycle of obesity and insulin resistance [17]. A major drawback of these agonists is their need for daily subcutaneous injections. However, long-acting GLP-1 analogues are currently in clinical development, allowing a once weekly application. In contrast, DPP-4 inhibitors can be taken orally resulting in an 80% reduction of DPP-4 activity and a 2-fold increase of circulating active GLP-1 peptide [18]. So far, sitagliptin, vildagliptin, and recently saxagliptin have been approved for clinical use. These drugs cause an HbA1c reduction of approximately 0.8%, but they have no impact on body weight [19].
While clinical outcome studies investigating the cardiovascular effects of GLP-1-based therapies have been initiated, results will not be available for a few years. However, a number of experimental and preliminary clinical data suggest a cardiovascular benefit of GLP-1-based therapies, which will be discussed in the following sections.
GLP-1 and the heart
GLP-1 and acute myocardial infarction
Cardioprotective effects of GLP-1 were first reported in patients with acute myocardial infarction who received 72 h of continuous GLP-1 infusion post angioplasty, leading to a significantly improved left ventricular systolic function [20]. This prompted a variety of additional studies investigating the therapeutic potential of GLP-1 in the context of myocardial ischemia.
While rapid revascularization of occluded vessels remains the main treatment strategy for myocardial infarction, additional approaches try to preserve tissue integrity by increasing the cellular tolerability to ischemia. Thereby, expansion of myocardial infarction depends on ischemic exposure and reperfusion injury caused by radical oxygen formation during revascularization. Experimental interventions applying fractional ischemia before vessel occlusion or gradual reperfusion after myocardial infarction can reduce tissue necrosis by approximately 50% [21]. Conceptually, this so called ischemic pre- or post-conditioning is used to identify new targets for pharmacological treatment [22]. Among these targets, the reperfusion injury salvage kinase (RISK) pathway has been identified as a promising candidate, together with the prosurvival signals phosphoinositide-3 (PI3) kinase, Akt, and extracellular signal-regulated kinase 1/2 (ERK1/2) [23]. Activation of the RISK pathway during reperfusion reduces myocardial infarct size by 40-50% [23]. Ongoing research is looking for RISK-modulating drugs with GLP-1 being of potential interest.
Cardioprotective effects of GLP-1 in acute myocardial ischemia have been described in a series of species and models [20, 24-36] although not confirmed in all studies [37, 38]. A substantial proportion of these investigations were done under ex-vivo conditions in isolated heart perfusion models [24, 27, 28, 31, 33-35], which successfully translated to short [20, 24-26, 29, 30, 32, 36] and longer lasting in vivo studies [27].
Administration of GLP-1 significantly reduced myocardial infarction size and led to a functional recovery of rodent heart perfusion models under ex vivo and in vivo conditions [24, 28, 31, 34]. Similarly, liraglutide prevented myocardial injury, improved survival in mice, and reduced post-infarction cardiac rupture [27]. This was also confirmed in DPP-4 knockout mice [39]. Furthermore, DPP-4 inhibition augmented myocardial function following myocardial infarction in rodent models [40, 41]. In rabbits, pre- or post-ischemic application of GLP-1 reduced infarction size, with best results seen when administered during reperfusion [25, 26]. These studies were extended to dogs, in which GLP-1 augmented regional wall motion recovery following a brief period of ischemia [26]. In pigs, exendin-4 limited infarct size and improved functional left ventricular recovery when given for 72 h starting with reperfusion [32]. However, two additional studies in pigs failed to demonstrate cardioprotective effects of GLP-1 or liraglutide in the context of acute myocardial infarction [37, 38]. This discrepancy may result from the use of different GLP-1 agonists or the timing of application prior to or post myocardial infarction.
At present, investigations in humans are limited. Besides the initial study, reporting the beneficial effects of GLP-1 in the context of acute myocardial infarction [20], two additional small studies have been conducted. The latter investigated the effects of continuous GLP-1 infusion in patients undergoing coronary artery bypass graft surgery. No effect of GLP-1 on myocardial contractility and hemodynamics was found in either study. Whereas, GLP-1 reduced the need for circulatory inotropic, or vasoactive, drug support following surgery [30, 42]. In a dobutamin stress test in patients with ischemic coronary artery disease, administration of GLP-1, or sitagliptin, improved myocardial wall motion [29, 36]. In conclusion, GLP-1 convincingly proved cardio-protective in ex-vivo and small animal models during acute ischemia. Results in large animal models remain conflicting, while studies in humans suggest cardioprotection, but they are not conclusive. Therefore, a prospective, double-blind clinical trial, investigating GLP-1 effectiveness in patients with acute myocardial infarction, remains very preferable. Also, it is not clear to what extent these direct GLP-1 effects can be found in patients treated with GLP-1 analogues or DPP-4 inhibitors.
Mechanisms of GLP-1-dependent cardioprotection during ischemia
Although GLP-1-dependent cardioprotection has been extensively studied, the relevant underlying mechanisms are still not fully understood. On cellular basis, GLP-1 was found to reduce cardiomyocyte apoptosis in response to ischemic/re-perfusion injury [24, 25, 27, 32, 43]. This can be attributed to GLP-1-dependent activation of PI3K, AKT, and ERK1/2, which together are known as the RISK pathway [24, 27, 32, 34, 43] (Figure 1). Furthermore, GLP-1 was found to activate the cell growth signaling cascade of mammalian target of rapamycin (mTOR) and p70s6K kinase, which are downstream of AKT and inhibit the proapoptotic protein BAD [24, 34, 43, 44]. However, this could not be confirmed in all models [27]. An additional antiapoptototic action of GLP-1 is attributable to the inhibition of GSK3β as another downstream target of the RISK pathway [27, 34, 43]. Finally, GLP-1 was found to increase the expression of redox sensitive transcription factors, namely nuclear factor (erythroid-derived 2)-like 2 (Nrf2) and peroxisome proliferator-activated receptor (PPAR) delta [27].
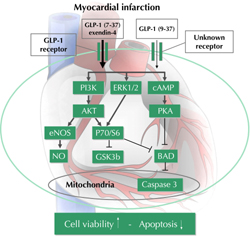 |
 |
Figure 1. Effects of GLP-1 during acute myocardial infarction. PI3K: phosphoinositol 3-kinase. ERK1/2: extracellular signal-regulated kinases. PKA: protein kinase A. eNOS: endothelial nitric oxide synthase. BAD: Bcl-2-associated death promoter. GSK: glycogen synthase kinase 3. |
|
Relevance of the GLP-1 receptor
Ongoing controversy debates the relevance of the known GLP-1 receptor as the target of GLP-1 actions in the cardiovascular system. This discussion is augmented by the persistent cardiovascular benefits associated with the GLP-1 metabolite (9-36), which does not activate the GLP-1 receptor [16].
Relevance of the GLP-1 receptor in the cardiovascular system has emerged through its broad expression in cardiomyocytes and endothelial cells, the decreased heart rate, elevated left ventricular (LV) end-diastolic pressure, and cardiac hypertrophy found in GLP-1 receptor knockout mice [33, 45]. It was also found that GLP-1 receptor knockout mice lose the liraglutide-dependent activation of prosurvival pathways in the heart [27]. Furthermore, GLP-1 receptor antagonist exendin-9 abolished the cardioprotective effects of GLP-1 in ischemia/reperfusion models. Similar effects were found with the inhibition of cAMP signaling as the downstream target of the GLP-1 receptor [24, 27, 28]. Also, in some experiments, simultaneous DPP-4 inhibition was required to reach GLP-1 effectiveness, suggesting that only the uncleaved GLP-1 (7-36) peptide was cardioprotective [24, 34]. This finding was further supported by observations of reduced myocardial infarction size, improved survival of DPP-4 knockout mice [39], and the augmented myocardial function seen with DPP-4 inhibition in rodent models [40, 41] and humans [29].
However, major relevance of the GLP-1 receptor has been challenged by other findings. Firstly, preserved GLP-1-mediated cardioprotection was found in hearts from GLP-1 receptor knockout mice [33]. Secondly, the GLP-1 metabolite (9-36)―which does not activate the GLP-1 receptor―was found to have infarct-sparing capacities similar to exendin-4 [35], although this was not confirmed in all studies [31, 33]. Interestingly, while exendin-4 lost its cell-protective effect in the absence of GLP-1 receptor, the effect was preserved for the GLP-1 metabolite [35]. This suggests the presence of an additional, yet unidentified, receptor only activated by the GLP-1 metabolite but not exendin-4 (Figure 1). However, this receptor seems to share significant structural and functional homology with the known GLP-1 receptor, as it was also found to increase cellular cAMP levels and was similarly antagonized by exendin-9 [35]. This suggests the presence of an additional G-protein-coupled receptor as target of the GLP-1 metabolite. Importantly, these observations question the use of exendin-9 as a tool to characterize specific effects of the known GLP-1 receptor.
GLP-1 and heart failure
GLP-1-dependent cardioprotection extends to the setting of chronic heart failure. This was firstly described in a dog model of pace maker-induced dilated cardiomyopathy. A continuous 48 h GLP-1 infusion improved cardiac function resulting in increased stroke volume and cardiac output, while simultaneously reducing heart rate, peripheral resistance, and plasma norepinephrine concentrations [46]. Similar effects were found in mouse models of primary dilated cardiomyopathy [47] and chronic ischemic [48], or metabolic, cardiomyopathy [49]. In humans, continuous GLP-1 infusion for 5 weeks augmented left ventricular ejection fraction and maximal oxygen uptake in a small study of patients with heart failures of classes NYHA III and IV [50]. In contrast, 48 h of GLP-1 infusion failed to improve cardiac function in a double-blind placebo-controlled crossover study in 20 patients with NYHA class II and III. This could have been due to the shorter application interval [51].
On molecular level, heart failure is characterized by a switch in primary substrate utilization from fatty acid oxidation in healthy subjects to glucose oxidation in heart failure patients [52] (Figure 2). The lower respiratory quotient of glucose thereby reduces myocardial oxygen demand [53]. This coping strategy is however defeated by a concomitant raise in local and systemic insulin resistance during heart failure, which limits glucose supply and causes a chronic state of energy deprivation [54]. Therefore, improvement of cardiomyocyte glucose delivery was identified as a target for heart failure therapy. GLP-1 directly increases cardiomyocyte glucose uptake, which happens independently of its insulinotropic actions and remains present under hyperinsulinemic clamp conditions [46]. Mechanistically, this might be attributable to increased GLP-1-dependent AKT activation and glucose transporter type 4 (GLUT4) translocation [47, 49]. It may also result from mitogen-activated protein (MAP) kinase and nitric oxide synthase 2 (NOS2)-dependent GLUT1 translocation [55]. Interestingly, in one study, the GLP-1 metabolite was also found to increase cardiomyocyte glucose uptake [11]. Additional cardioprotective effects of GLP-1 might result from a reduction of oxidative stress, reduced mitochondrial density, and increased UCP3 expression [55].
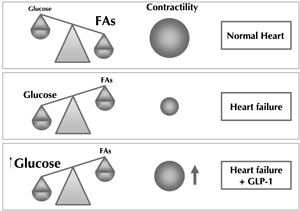 |
 |
Figure 2. Modulation of myocardial substrate utilization by GLP-1 during heart failure. FA: fatty acids. |
|
GLP-1 and the vessel wall
GLP-1 has vasoprotective effects. GLP-1-dependent improvement of vascular function has been reported in healthy subjects and type 2 diabetic patients with stable coronary artery disease [56, 57]. Continuous infusion of the peptide thereby rapidly increased acetylcholine, or flow-mediated, vasodilatation of the brachial artery under hyperinsulinemic clamp conditions, demonstrating a direct and insulin-independent effect [56, 57]. Similar results were obtained from different experimental models in which GLP-1 increased vasorelaxation in coronary or pulmonary arteries in a cAMP- and endothelial nitric oxide synthase (eNOS)-dependent manner [33, 58]. In contrast, other findings suggested vascular smooth muscle to be of primary relevance for vasorelaxation in an eNOS-independent manner [59]. Importantly, eNOS inhibition abrogated 50% of the cardioprotective effect of GLP-1 following myocardial infarction, demonstrating a relevant contribution of the vascular system to GLP-1-dependent cardioprotection [33]. Interestingly, DPP-4 inhibition was able to abrogate GLP-1-dependent vasorelaxation, while this was preserved for the GLP-1 metabolite, even in the absence of the GLP-1 receptor [33]. This suggests that the GLP-1 metabolite was the primary cause of vasorelaxation. However, others reported similar improvement to endothelial function with exendin-4 as a non-cleavable peptide and classical activator of the known GLP-1 receptor [60].
The vasorelaxative effects of GLP-1 might in part explain the antihypertensive potential demonstrated in long-term clinical trails using exenatide, liraglutide, or DPP-4 inhibitors [61-63], although most of the antihypertensive effects may be due to weight loss. Consistently, a 2-week GLP-1 treatment reduced blood pressure and proteinuria in salt-sensitive hypertensive rats. This effect was paralleled by increased natriuresis and improved endothelial function [64]. However, these observations contrast to heart rate- and blood pressure-increasing effects of short-term GLP-1 application, as found by others [65, 66]. Potentially, this reflects a GLP-1-dependent bidirectional modulation of blood pressure in a time-dependent manner [67].
Additional vasoprotective effects of GLP-1 have been described in acute vascular injury models in which exendin-4 reduced neointima formation and smooth muscle cell proliferation after wire injury [68]. Furthermore, chronic treatment with exendin-4 reduced atherosclerotic lesion formation in apolipoprotein E (ApoE)-deficient mice on a high fat diet [69]. Both studies reported reduced vascular inflammation under GLP-1 agonist treatment which was attributed to a variety of immune and vascular cells (Figure 3). GLP-1 was found to (i) reduce lipopolysaccharide (LPS)-dependent cytokine release from macrophages, (ii) inhibit the migration of T cells, (iii) repress endothelial cell adhesion molecule expression, and (iv) impair vascular smooth muscle cell proliferation [69-74]. These observations translated to a reduced mortality of exendin-4-treated mice challenged with LPS [75]. In macrophages, anti-inflammatory effects of GLP-1 have been attributed to inhibition of NF-κB in a cAMP- and protein kinase A (PKA)-dependent manner [69]. Similarly, GLP-1 was found to inhibit NF-κB in endothelial cells, although mediated by AMP-activated protein kinase (AMPK) [74]. However, neither cAMP, Akt, nor mitogen-activated protein kinase (MAPK) signaling was found to be responsible for antiproliferative effects by GLP-1 seen in vascular smooth muscle cells [68]. Anti-inflammatory effects of GLP-1 might also beneficially modulate the coagulation system, with decreased expression of plasminogen activator inhibitor being found in response to liraglutide in endothelial cells [72].
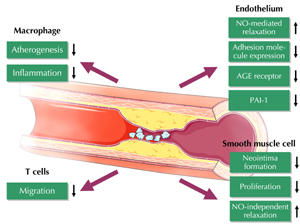 |
 |
Figure 3. Vasoprotective effects of GLP-1 |
|
It was also found that GLP-1 can down-regulate the receptor for advanced glycation end-products (RAGE) in endothelial cells, which contributes to hyperglycemia-induced vascular inflammation in diabetes [71, 76]. Consequently, generation of reactive oxidant species in response to advanced glycation end product (AGE) exposure was reduced by GLP-1 agonist treatment [71]. Interestingly, similar anti-inflammatory and RAGE suppressive effects were reported for DPP-4 inhibition in diabetic rats resulting in reduced NF-κB activation [77]. These anti-inflammatory actions might also explain the improved survival of endotoxin-challenged rats when treated with DPP-4 inhibitors [75].
Furthermore, GLP-1 agonists have been found to improve lipid metabolism during longer term treatment intervals, leading to a reduction of triglyceride levels and increased HDL cholesterol [78, 79]. This effect seems to be due to a GLP-1-dependent improvement of postprandial lipemia [80], although it might also largely be secondary to improved glucose metabolism and body weight reduction.
The described effects on heart and vascular system, including the modulation of risk factors, might translate into long-term improvement in clinical prognosis. Indeed, a large retrospective database analysis recently compared cardiovascular events in diabetic patients treated since 2005 with exendin-4 or other antidiabetic drugs. Patients receiving exendin-4 were more likely to present with ischemic heart disease, obesity, hypertension, and/or other co-morbidities. However, the use of exendin-4 was associated with a 19% reduction in CV-events and a 12% reduction in CVD-related hospitalization, suggesting a cardiovascular benefits of GLP-1-based therapies [81].
Conclusions
Current experimental and early clinical data suggest that GLP-1-based therapies may modulate vascular and cardiac function. As such, they exhibit protective effects in the cardiovascular system. Moreover, retrospective analyses of phase II and III studies with GLP-1 analogues and DPP-4 inhibitors showed a trend towards reduced cardiovascular events compared to placebo or comparator. This finding raised the hypothesis that these beneficial effects may translate into a reduction in cardiovascular morbidity and mortality in treated patients. Large randomized, prospective cardiovascular outcome trials are currently under way for various GLP-1-based drugs. The anxiously awaited results will clarify whether these therapies have the potential to reduce cardiovascular risk in patients with diabetes.
Disclosures (conflict of interests statement): ML lectures for Novo Nordisk and MSD. NM receives funding from Boehringer Ingelheim and MSD, is advisory board member of Boehringer Ingelheim, MSD, GSK, Astra Zeneca, BMS, and Sanofi-Aventis, and lectures for Boehringer Ingelheim, Novo Nordisk, MSD, GSK, Astra Zeneca, BMS, Lilly, and Berlin Chemie.
References
- Brown A, Reynolds LR, Bruemmer D. Intensive glycemic control and cardiovascular disease: an update. Nat Rev Cardiol 2010. 7(7):369-375. [DOD] [CrossRef]
- Effect of intensive blood-glucose control with metformin on complications in overweight patients with type 2 diabetes (UKPDS 34). UK Prospective Diabetes Study (UKPDS) Group. Lancet 1998. 352(9131):854-865. [DOD] [CrossRef]
- Schramm TK, Gislason GH, Vaag A, Rasmussen JN, Folke F, Hansen ML, Fosbol EL, Kober L, Norgaard ML, Madsen M, et al. Mortality and cardiovascular risk associated with different insulin secretagogues compared with metformin in type 2 diabetes, with or without a previous myocardial infarction: a nationwide study. Eur Heart J 2011. In press. [DOD]
- Simpson SH, Majumdar SR, Tsuyuki RT, Eurich DT, Johnson JA. Dose-response relation between sulfonylurea drugs and mortality in type 2 diabetes mellitus: a population-based cohort study. CMAJ 2006. 174(2):169-174. [DOD] [CrossRef]
- Tzoulaki I, Molokhia M, Curcin V, Little MP, Millett CJ, Ng A, Hughes RI, Khunti K, Wilkins MR, Majeed A, et al. Risk of cardiovascular disease and all cause mortality among patients with type 2 diabetes prescribed oral antidiabetes drugs: retrospective cohort study using UK general practice research database. BMJ 2009. 339:b4731. [DOD] [CrossRef]
- Ginsberg H, Plutzky J, Sobel BE. A review of metabolic and cardiovascular effects of oral antidiabetic agents: beyond glucose-level lowering. J Cardiovasc Risk 1999. 6(5):337-346. [DOD]
- Baggio LL, Drucker DJ. Biology of incretins: GLP-1 and GIP. Gastroenterology 2007. 132(6):2131-2157. [DOD] [CrossRef]
- Meier JJ, Nauck MA. Glucagon-like peptide 1 (GLP-1) in biology and pathology. Diabetes Metab Res Rev 2005. 21(2):91-117. [DOD] [CrossRef]
- Mojsov S, Heinrich G, Wilson IB, Ravazzola M, Orci L, Habener JF. Preproglucagon gene expression in pancreas and intestine diversifies at the level of post-translational processing. J Biol Chem 1986. 261(25):11880-11889. [DOD]
- Orskov C, Wettergren A, Holst JJ. Biological effects and metabolic rates of glucagonlike peptide-1 7-36 amide and glucagonlike peptide-1 7-37 in healthy subjects are indistinguishable. Diabetes 1993. 42(5):658-661. [DOD] [CrossRef]
- Nikolaidis LA, Elahi D, Shen YT, Shannon RP. Active metabolite of GLP-1 mediates myocardial glucose uptake and improves left ventricular performance in conscious dogs with dilated cardiomyopathy. Am J Physiol Heart Circ Physiol 2005. 289(6):H2401-H2408. [DOD] [CrossRef]
- Deacon CF, Johnsen AH, Holst JJ. Degradation of glucagon-like peptide-1 by human plasma in vitro yields an N-terminally truncated peptide that is a major endogenous metabolite in vivo. J Clin Endocrinol Metab 1995. 80(3):952-957. [DOD] [CrossRef]
- Knudsen LB, Pridal L. Glucagon-like peptide-1-(9-36) amide is a major metabolite of glucagon-like peptide-1-(7-36) amide after in vivo administration to dogs, and it acts as an antagonist on the pancreatic receptor. Eur J Pharmacol 1996. 318(2-3):429-435. [DOD] [CrossRef]
- Vahl TP, Paty BW, Fuller BD, Prigeon RL, D'Alessio DA. Effects of GLP-1-(7-36)NH2, GLP-1-(7-37), and GLP-1-(9-36)NH2 on intravenous glucose tolerance and glucose-induced insulin secretion in healthy humans. J Clin Endocrinol Metab 2003. 88(4):1772-1779. [DOD] [CrossRef]
- Deacon CF, Plamboeck A, Moller S, Holst JJ. GLP-1-(9-36) amide reduces blood glucose in anesthetized pigs by a mechanism that does not involve insulin secretion. Am J Physiol Endocrinol Metab 2002. 282(4):E873-E879. [DOD]
- Abu-Hamdah R, Rabiee A, Meneilly GS, Shannon RP, Andersen DK, Elahi D. The extrapancreatic effects of glucagon-like peptide-1 and related peptides. J Clin Endocrinol Metab 2009. 94(6):1843-1852. [DOD] [CrossRef]
- Nauck MA. Incretin-based therapies for type 2 diabetes mellitus: properties, functions, and clinical implications. Am J Med 2011. 124(1 Suppl):S3-S18. [DOD] [CrossRef]
- Deacon CF, Carr RD, Holst JJ. DPP-4 inhibitor therapy: new directions in the treatment of type 2 diabetes. Front Biosci 2008. 13:1780-1794. [DOD] [CrossRef]
- Ahren B. Clinical results of treating type 2 diabetic patients with sitagliptin, vildagliptin or saxagliptin - diabetes control and potential adverse events. Best Pract Res Clin Endocrinol Metab 2009. 23(4):487-498. [DOD] [CrossRef]
- Nikolaidis LA, Mankad S, Sokos GG, Miske G, Shah A, Elahi D, Shannon RP. Effects of glucagon-like peptide-1 in patients with acute myocardial infarction and left ventricular dysfunction after successful reperfusion. Circulation 2004. 109(8):962-965. [DOD] [CrossRef]
- Hausenloy DJ, Yellon DM. Preconditioning and postconditioning: underlying mechanisms and clinical application. Atherosclerosis 2009. 204(2):334-341. [DOD] [CrossRef]
- Ovize M, Baxter GF, Di Lisa F, Ferdinandy P, Garcia-Dorado D, Hausenloy DJ, Heusch G, Vinten-Johansen J, Yellon DM, Schulz R. Postconditioning and protection from reperfusion injury: where do we stand? Position paper from the Working Group of Cellular Biology of the Heart of the European Society of Cardiology. Cardiovasc Res 2010. 87(3):406-423. [DOD] [CrossRef]
- Hausenloy DJ, Yellon DM. New directions for protecting the heart against ischaemia-reperfusion injury: targeting the Reperfusion Injury Salvage Kinase (RISK)-pathway. Cardiovasc Res 2004. 61(3):448-460. [DOD] [CrossRef]
- Bose AK, Mocanu MM, Carr RD, Brand CL, Yellon DM. Glucagon-like peptide 1 can directly protect the heart against ischemia/reperfusion injury. Diabetes 2005. 54(1):146-151. [DOD] [CrossRef]
- Matsubara M, Kanemoto S, Leshnower BG, Albone EF, Hinmon R, Plappert T, Gorman JH 3rd, Gorman RC. Single dose GLP-1-Tf ameliorates myocardial ischemia/reperfusion injury. J Surg Res 2011. 165(1):38-45. [DOD] [CrossRef]
- Nikolaidis LA, Doverspike A, Hentosz T, Zourelias L, Shen YT, Elahi D, Shannon RP. Glucagon-like peptide-1 limits myocardial stunning following brief coronary occlusion and reperfusion in conscious canines. J Pharmacol Exp Ther 2005. 312(1):303-308. [DOD] [CrossRef]
- Noyan-Ashraf MH, Momen MA, Ban K, Sadi AM, Zhou YQ, Riazi AM, Baggio LL, Henkelman RM, Husain M, Drucker DJ. GLP-1R agonist liraglutide activates cytoprotective pathways and improves outcomes after experimental myocardial infarction in mice. Diabetes 2009. 58(4):975-983. [DOD] [CrossRef]
- Ossum A, van Deurs U, Engstrom T, Jensen JS, Treiman M. The cardioprotective and inotropic components of the postconditioning effects of GLP-1 and GLP-1(9-36)a in an isolated rat heart. Pharmacol Res 2009. 60(5):411-417. [DOD] [CrossRef]
- Read PA, Khan FZ, Heck PM, Hoole SP, Dutka DP. DPP-4 inhibition by sitagliptin improves the myocardial response to dobutamine stress and mitigates stunning in a pilot study of patients with coronary artery disease. Circ Cardiovasc Imaging 2010. 3(2):195-201. [DOD] [CrossRef]
- Sokos GG, Bolukoglu H, German J, Hentosz T, Magovern GJ Jr, Maher TD, Dean DA, Bailey SH, Marrone G, Benckart DH, et al. Effect of glucagon-like peptide-1 (GLP-1) on glycemic control and left ventricular function in patients undergoing coronary artery bypass grafting. Am J Cardiol 2007. 100(5):824-829. [DOD] [CrossRef]
- Sonne DP, Engstrom T, Treiman M. Protective effects of GLP-1 analogues exendin-4 and GLP-1(9-36) amide against ischemia-reperfusion injury in rat heart. Regul Pept 2008. 146(1-3):243-249. [DOD] [CrossRef]
- Timmers L, Henriques JP, de Kleijn DP, Devries JH, Kemperman H, Steendijk P, Verlaan CW, Kerver M, Piek JJ, Doevendans PA, et al. Exenatide reduces infarct size and improves cardiac function in a porcine model of ischemia and reperfusion injury. J Am Coll Cardiol 2009. 53(6):501-510. [DOD] [CrossRef]
- Ban K, Noyan-Ashraf MH, Hoefer J, Bolz SS, Drucker DJ, Husain M. Cardioprotective and vasodilatory actions of glucagon-like peptide 1 receptor are mediated through both glucagon-like peptide 1 receptor-dependent and -independent pathways. Circulation 2008. 117(18):2340-2350. [DOD] [CrossRef]
- Bose AK, Mocanu MM, Carr RD, Yellon DM. Myocardial ischaemia-reperfusion injury is attenuated by intact glucagon like peptide-1 (GLP-1) in the in vitro rat heart and may involve the p70s6K pathway. Cardiovasc Drugs Ther 2007. 21(4):253-256. [DOD] [CrossRef]
- Ban K, Kim KH, Cho CK, Sauve M, Diamandis EP, Backx PH, Drucker DJ, Husain M. Glucagon-like peptide (GLP)-1(9-36)amide-mediated cytoprotection is blocked by exendin(9-39) yet does not require the known GLP-1 receptor. Endocrinology 2010. 151(4):1520-1531. [DOD] [CrossRef]
- Read PA, Khan FZ, Dutka DP. Cardioprotection against ischaemia induced by dobutamine stress using glucagon-like peptide-1 in patients with coronary artery disease. Heart 2011. In press. [DOD]
- Kavianipour M, Ehlers MR, Malmberg K, Ronquist G, Ryden L, Wikstrom G, Gutniak M. Glucagon-like peptide-1 (7-36) amide prevents the accumulation of pyruvate and lactate in the ischemic and non-ischemic porcine myocardium. Peptides 2003. 24(4):569-578. [DOD] [CrossRef]
- Kristensen J, Mortensen UM, Schmidt M, Nielsen PH, Nielsen TT, Maeng M. Lack of cardioprotection from subcutaneously and preischemic administered liraglutide in a closed chest porcine ischemia reperfusion model. BMC Cardiovasc Disord 2009. 9:31. [DOD] [CrossRef]
- Sauve M, Ban K, Momen MA, Zhou YQ, Henkelman RM, Husain M, Drucker DJ. Genetic deletion or pharmacological inhibition of dipeptidyl peptidase-4 improves cardiovascular outcomes after myocardial infarction in mice. Diabetes 2010. 59(4):1063-1073. [DOD] [CrossRef]
- Huisamen B, Genis A, Marais E, Lochner A. Pre-treatment with a DPP-4 inhibitor is infarct sparing in hearts from obese, pre-diabetic rats. Cardiovasc Drugs Ther 2011. 25(1):13-20. [DOD] [CrossRef]
- Ye Y, Keyes KT, Zhang C, Perez-Polo JR, Lin Y, Birnbaum Y. The myocardial infarct size-limiting effect of sitagliptin is PKA-dependent, whereas the protective effect of pioglitazone is partially dependent on PKA. Am J Physiol Heart Circ Physiol 2010. 298(5):H1454-H1465. [DOD] [CrossRef]
- Mussig K, Oncu A, Lindauer P, Heininger A, Aebert H, Unertl K, Ziemer G, Haring HU, Holst JJ, Gallwitz B. Effects of intravenous glucagon-like peptide-1 on glucose control and hemodynamics after coronary artery bypass surgery in patients with type 2 diabetes. Am J Cardiol 2008. 102(5):646-647. [DOD] [CrossRef]
- Ravassa S, Zudaire A, Carr RD, Diez J. Antiapoptotic effects of GLP-1 in murine HL-1 cardiomyocytes. Am J Physiol Heart Circ Physiol 2011. 300(4):H1361-H1372. [DOD] [CrossRef]
- Quoyer J, Longuet C, Broca C, Linck N, Costes S, Varin E, Bockaert J, Bertrand G, Dalle S. GLP-1 mediates antiapoptotic effect by phosphorylating Bad through a beta-arrestin 1-mediated ERK1/2 activation in pancreatic beta-cells. J Biol Chem 2010. 285(3):1989-2002. [DOD] [CrossRef]
- Gros R, You X, Baggio LL, Kabir MG, Sadi AM, Mungrue IN, Parker TG, Huang Q, Drucker DJ, Husain M. Cardiac function in mice lacking the glucagon-like peptide-1 receptor. Endocrinology 2003. 144(6):2242-2252. [DOD] [CrossRef]
- Nikolaidis LA, Elahi D, Hentosz T, Doverspike A, Huerbin R, Zourelias L, Stolarski C, Shen YT, Shannon RP. Recombinant glucagon-like peptide-1 increases myocardial glucose uptake and improves left ventricular performance in conscious dogs with pacing-induced dilated cardiomyopathy. Circulation 2004. 110(8):955-961. [DOD] [CrossRef]
- Vyas AK, Yang KC, Woo D, Tzekov A, Kovacs A, Jay PY, Hruz PW. Exenatide improves glucose homeostasis and prolongs survival in a murine model of dilated cardiomyopathy. PLoS One 2011. 6(2):e17178. [DOD] [CrossRef]
- Liu Q, Anderson C, Broyde A, Polizzi C, Fernandez R, Baron A, Parkes DG. Glucagon-like peptide-1 and the exenatide analogue AC3174 improve cardiac function, cardiac remodeling, and survival in rats with chronic heart failure. Cardiovasc Diabetol 2010. 9:76. [DOD] [CrossRef]
- Poornima I, Brown SB, Bhashyam S, Parikh P, Bolukoglu H, Shannon RP. Chronic glucagon-like peptide-1 infusion sustains left ventricular systolic function and prolongs survival in the spontaneously hypertensive, heart failure-prone rat. Circ Heart Fail 2008. 1(3):153-160. [DOD] [CrossRef]
- Sokos GG, Nikolaidis LA, Mankad S, Elahi D, Shannon RP. Glucagon-like peptide-1 infusion improves left ventricular ejection fraction and functional status in patients with chronic heart failure. J Card Fail 2006. 12(9):694-699. [DOD] [CrossRef]
- Halbirk M, Norrelund H, Moller N, Holst JJ, Schmitz O, Nielsen R, Nielsen-Kudsk JE, Nielsen SS, Nielsen TT, Eiskjaer H, et al. Cardiovascular and metabolic effects of 48-h glucagon-like peptide-1 infusion in compensated chronic patients with heart failure. Am J Physiol Heart Circ Physiol 2010. 298(3):H1096-H1102. [DOD] [CrossRef]
- Lopaschuk GD, Ussher JR, Folmes CD, Jaswal JS, Stanley WC. Myocardial fatty acid metabolism in health and disease. Physiol Rev 2010. 90(1):207-258. [DOD] [CrossRef]
- Lee L, Horowitz J, Frenneaux M. Metabolic manipulation in ischaemic heart disease, a novel approach to treatment. Eur Heart J 2004. 25(8):634-641. [DOD] [CrossRef]
- Nikolaidis LA, Sturzu A, Stolarski C, Elahi D, Shen YT, Shannon RP. The development of myocardial insulin resistance in conscious dogs with advanced dilated cardiomyopathy. Cardiovasc Res 2004. 61(2):297-306. [DOD] [CrossRef]
- Bhashyam S, Fields AV, Patterson B, Testani JM, Chen L, Shen YT, Shannon RP. Glucagon-like peptide-1 increases myocardial glucose uptake via p38alpha MAP kinase-mediated, nitric oxide-dependent mechanisms in conscious dogs with dilated cardiomyopathy. Circ Heart Fail 2010. 3(4):512-521. [DOD] [CrossRef]
- Basu A, Charkoudian N, Schrage W, Rizza RA, Basu R, Joyner MJ. Beneficial effects of GLP-1 on endothelial function in humans: dampening by glyburide but not by glimepiride. Am J Physiol Endocrinol Metab 2007. 293(5):E1289-E1295. [DOD] [CrossRef]
- Nystrom T, Gutniak MK, Zhang Q, Zhang F, Holst JJ, Ahren B, Sjoholm A. Effects of glucagon-like peptide-1 on endothelial function in type 2 diabetes patients with stable coronary artery disease. Am J Physiol Endocrinol Metab 2004. 287(6):E1209-E1215. [DOD] [CrossRef]
- Richter G, Feddersen O, Wagner U, Barth P, Goke R, Goke B. GLP-1 stimulates secretion of macromolecules from airways and relaxes pulmonary artery. Am J Physiol 1993. 265(4 Pt 1):L374-L381. [DOD]
- Nystrom T, Gonon AT, Sjoholm A, Pernow J. Glucagon-like peptide-1 relaxes rat conduit arteries via an endothelium-independent mechanism. Regul Pept 2005. 125(1-3):173-177. [DOD] [CrossRef]
- Green BD, Hand KV, Dougan JE, McDonnell BM, Cassidy RS, Grieve DJ. GLP-1 and related peptides cause concentration-dependent relaxation of rat aorta through a pathway involving KATP and cAMP. Arch Biochem Biophys 2008. 478(2):136-142. [DOD] [CrossRef]
- Garber A, Henry R, Ratner R, Garcia-Hernandez PA, Rodriguez-Pattzi H, Olvera-Alvarez I, Hale PM, Zdravkovic M, Bode B. Liraglutide versus glimepiride monotherapy for type 2 diabetes (LEAD-3 Mono): a randomised, 52-week, phase III, double-blind, parallel-treatment trial. Lancet 2009. 373(9662):473-481. [DOD] [CrossRef]
- Moretto TJ, Milton DR, Ridge TD, Macconell LA, Okerson T, Wolka AM, Brodows RG. Efficacy and tolerability of exenatide monotherapy over 24 weeks in antidiabetic drug-naive patients with type 2 diabetes: a randomized, double-blind, placebo-controlled, parallel-group study. Clin Ther 2008. 30(8):1448-1460. [DOD] [CrossRef]
- Ogawa S, Ishiki M, Nako K, Okamura M, Senda M, Mori T, Ito S. Sitagliptin, a dipeptidyl peptidase-4 inhibitor, decreases systolic blood pressure in Japanese hypertensive patients with type 2 diabetes. Tohoku J Exp Med 2011. 223(2):133-135. [DOD] [CrossRef]
- Yu M, Moreno C, Hoagland KM, Dahly A, Ditter K, Mistry M, Roman RJ. Antihypertensive effect of glucagon-like peptide 1 in Dahl salt-sensitive rats. J Hypertens 2003. 21(6):1125-1135. [DOD] [CrossRef]
- Yamamoto H, Lee CE, Marcus JN, Williams TD, Overton JM, Lopez ME, Hollenberg AN, Baggio L, Saper CB, Drucker DJ, et al. Glucagon-like peptide-1 receptor stimulation increases blood pressure and heart rate and activates autonomic regulatory neurons. J Clin Invest 2002. 110(1):43-52. [DOD]
- Barragan JM, Eng J, Rodriguez R, Blazquez E. Neural contribution to the effect of glucagon-like peptide-1-(7-36) amide on arterial blood pressure in rats. Am J Physiol 1999. 277(5 Pt 1):E784-E791. [DOD]
- Bojanowska E, Stempniak B. Effects of centrally or systemically injected glucagon-like peptide-1 (7-36) amide on release of neurohypophysial hormones and blood pressure in the rat. Regul Pept 2000. 91(1-3):75-81. [DOD] [CrossRef]
- Goto H, Nomiyama T, Mita T, Yasunari E, Azuma K, Komiya K, Arakawa M, Jin WL, Kanazawa A, Kawamori R, et al. Exendin-4, a glucagon-like peptide-1 receptor agonist, reduces intimal thickening after vascular injury. Biochem Biophys Res Commun 2011. 405(1):79-84. [DOD] [CrossRef]
- Arakawa M, Mita T, Azuma K, Ebato C, Goto H, Nomiyama T, Fujitani Y, Hirose T, Kawamori R, Watada H. Inhibition of monocyte adhesion to endothelial cells and attenuation of atherosclerotic lesion by a glucagon-like peptide-1 receptor agonist, exendin-4. Diabetes 2010. 59(4):1030-1037. [DOD] [CrossRef]
- Marx N, Burgmaier M, Heinz P, Ostertag M, Hausauer A, Bach H, Durst R, Hombach V, Walcher D. Glucagon-like peptide-1(1-37) inhibits chemokine-induced migration of human CD4-positive lymphocytes. Cell Mol Life Sci 2010. 67(20):3549-3555. [DOD] [CrossRef]
- Ishibashi Y, Nishino Y, Matsui T, Takeuchi M, Yamagishi SI. Glucagon-like peptide-1 suppresses advanced glycation end product-induced monocyte chemoattractant protein-1 expression in mesangial cells by reducing advanced glycation end product receptor level. Metabolism 2011. In press. [DOD]
- Liu H, Hu Y, Simpson RW, Dear AE. Glucagon-like peptide-1 attenuates tumour necrosis factor-alpha-mediated induction of plasminogen (corrected) activator inhibitor-1 expression. J Endocrinol 2008. 196(1):57-65. [DOD] [CrossRef]
- Liu H, Dear AE, Knudsen LB, Simpson RW. A long-acting glucagon-like peptide-1 analogue attenuates induction of plasminogen activator inhibitor type-1 and vascular adhesion molecules. J Endocrinol 2009. 201(1):59-66. [DOD] [CrossRef]
- Hattori Y, Jojima T, Tomizawa A, Satoh H, Hattori S, Kasai K, Hayashi T. A glucagon-like peptide-1 (GLP-1) analogue, liraglutide, upregulates nitric oxide production and exerts anti-inflammatory action in endothelial cells. Diabetologia 2010. 53(10):2256-2263. [DOD] [CrossRef]
- Ku HC, Chen WP, Su MJ. GLP-1 signaling preserves cardiac function in endotoxemic Fischer 344 and DPP4-deficient rats. Naunyn Schmiedebergs Arch Pharmacol 2010. 382(5-6):463-474. [DOD] [CrossRef]
- Oeseburg H, de Boer RA, Buikema H, van der Harst P, van Gilst WH, Sillje HH. Glucagon-like peptide 1 prevents reactive oxygen species-induced endothelial cell senescence through the activation of protein kinase A. Arterioscler Thromb Vasc Biol 2010. 30(7):1407-1414. [DOD] [CrossRef]
- Matsui T, Nishino Y, Takeuchi M, Yamagishi S. Vildagliptin blocks vascular injury in thoracic aorta of diabetic rats by suppressing advanced glycation end product-receptor axis. Pharmacol Res 2011. 63(5):383-388. [DOD] [CrossRef]
- Zinman B, Gerich J, Buse JB, Lewin A, Schwartz S, Raskin P, Hale PM, Zdravkovic M, Blonde L. Efficacy and safety of the human glucagon-like peptide-1 analog liraglutide in combination with metformin and thiazolidinedione in patients with type 2 diabetes (LEAD-4 Met+TZD). Diabetes Care 2009. 32(7):1224-1230. [DOD] [CrossRef]
- Buse JB, Rosenstock J, Sesti G, Schmidt WE, Montanya E, Brett JH, Zychma M, Blonde L. Liraglutide once a day versus exenatide twice a day for type 2 diabetes: a 26-week randomised, parallel-group, multinational, open-label trial (LEAD-6). Lancet 2009. 374(9683):39-47. [DOD] [CrossRef]
- Meier JJ, Gethmann A, Gotze O, Gallwitz B, Holst JJ, Schmidt WE, Nauck MA. Glucagon-like peptide 1 abolishes the postprandial rise in triglyceride concentrations and lowers levels of non-esterified fatty acids in humans. Diabetologia 2006. 49(3):452-458. [DOD] [CrossRef]
- Best JH, Hoogwerf BJ, Herman WH, Pelletier EM, Smith DB, Wenten M, Hussein MA. Risk of cardiovascular disease events in patients with type 2 diabetes prescribed the glucagon-like peptide 1 (GLP-1) receptor agonist exenatide twice daily or other glucose-lowering therapies: a retrospective analysis of the LifeLink database. Diabetes Care 2011. 34(1):90-95. [DOD] [CrossRef]
This article has been cited by other articles:
|
PEGylated exendin-4, a modified GLP-1 analog exhibits more potent cardioprotection than its unmodified parent molecule on a dose to dose basis in a murine model of myocardial infarction
Sun Z, Tong G, Kim TH, Ma N, Niu G, Cao F, Chen X
Theranostics 2015. 5(3):240-250
|
|
|
Optimising cardioprotection during myocardial ischaemia: targeting potential intracellular pathways with glucagon-like peptide-1
Clarke SJ, McCormick LM, Dutka DP
Cardiovasc Diabetol 2014. 13:12
|
|
|
Pleiotropic effects of incretins and antidiabetic drugs with incretine mechanism
Winkler G
Orv Hetil 2013. 154(7):248-255
|
|
|
The dipeptidyl peptidase-4 inhibitor des-fluoro-sitagliptin regulates brown adipose tissue uncoupling protein levels in mice with diet-induced obesity
Shimasaki T, Masaki T, Mitsutomi K, Ueno D, Gotoh K, Chiba S, Kakuma T, Yoshimatsu H
Plos One 2013. 8(5):e63626
|
|
|
Physiology of incretins and loss of incretin effect in type 2 diabetes and obesity
Opinto G, Natalicchio A, Marchetti P
Arch Physiol Biochem 2013. 119(4):170-178
|
|
|
Glucagon-like peptide-1 (GLP-1) and its split products GLP-1(9-37) and GLP-1(28-37) stabilize atherosclerotic lesions in apoe⁻/⁻ mice
Burgmaier M, Liberman A, Möllmann J, Kahles F, Reith S, Lebherz C, Marx N, Lehrke M
Atherosclerosis 2013. 231(2):427-435
|
|
|
Circulating concentrations of GLP-1 are associated with coronary atherosclerosis in humans
Piotrowski K, Becker M, Zugwurst J, Biller-Friedmann I, Spoettl G, Greif M, Leber AW, Becker A, Laubender RP, Lebherz C, Goeke B, Marx N, Parhofer KG, Lehrke M
Cardiovasc Diabetol 2013. 12:117
|
|
|
New antidiabetic therapies: innovative strategies for an old problem
Lehrke M, Marx N
Curr Opin Lipidol 2012. 23(6):569-575
|
|
|
Physiology of incretins in health and disease
Deacon CF, Ahren B
Rev Diabet Stud 2011. 8(3):293-306
|
|
|
The continuing need for drug development and clinical trials in type 2 diabetes and its complications: introduction to the RDS Special Issue
Raz I, Gallwitz B
Rev Diabet Stud 2011. 8(3):288-292
|
|
|