Review
Rev Diabet Stud,
2017,
14(1):51-78 |
DOI 10.1900/RDS.2017.14.51 |
Encapsulated Islet Transplantation: Where Do We Stand?
Vijayaganapathy Vaithilingam1,2, Sumeet Bal1, Bernard E. Tuch3
1Materials Science and Engineering, Commonwealth Scientific and Industrial Research Organization (CSIRO), North Ryde, New South Wales, Australia
2Romvijay Biootech Private Limited, Kanniakoil, Pondicherry, India
3School of Medical Sciences, The University of Sydney, Sydney, New South Wales, Australia
Address correspondence to: Vijayaganapathy Vaithilingam, email: vvijayaganapathy@gmail.com
Manuscript submitted June 11, 2017; accepted June 11, 2017.
Keywords: diabetes, beta-cell, isletencapsulation, pericapsular fibrosis, nanoencapsulation, stem cells
Abstract
Transplantation of pancreatic islets encapsulated within immuno-protective microcapsules is a strategy that has the potential to overcome graft rejection without the need for toxic immunosuppressive medication. However, despite promising preclinical studies, clinical trials using encapsulated islets have lacked long-term efficacy, and although generally considered clinically safe, have not been encouraging overall. One of the major factors limiting the long-term function of encapsulated islets is the host's immunological reaction to the transplanted graft which is often manifested as pericapsular fibrotic overgrowth (PFO). PFO forms a barrier on the capsule surface that prevents the ingress of oxygen and nutrients leading to islet cell starvation, hypoxia and death. The mechanism of PFO formation is still not elucidated fully, and studies using a pig model have tried to understand the host immune response to empty alginate microcapsules. In this review, the varied strategies to overcome or reduce PFO are discussed, including alginate purification, altering microcapsule geometry, modifying alginate chemical composition, co-encapsulation with immunomodulatory cells, administration of pharmacological agents, and alternative transplantation sites. Nanoencapsulation technologies, such as conformal and layer-by-layer coating technologies, as well as nanofiber, thin-film nanoporous devices, and silicone-based NanoGland devices are also addressed. Finally, this review outlines recent progress in imaging technologies to track encapsulated cells, as well as promising perspectives concerning the production of insulin-producing cells from stem cells for encapsulation.
Abbreviations: APA alginate-poly-l-lysine-alginate; BGL blood glucose level; CITR Collaborative Islet Transplant Registry; CT computed tomography; ECM extracellular matrix; FGF fibroblast growth factor; GAD glutamic acid decarboxylase; hESC human embryonic stem cells; HMGB1 high-mobility group box 1; IBMIR instant blood-mediated immune response; IDD insulin-dependent diabetes; IGF insulin-like growth factor; IL-1beta interleukin -1 beta; iPSC induced pluripotent stem cells; LPS lipopolysaccharide; MPEG methoxy poly ethylene glycol; MRI magnetic resonance imaging; MSC mesenchymal stem cells; NEED nanofiber-enabled encapsulation devices; PAMP pathogen-associated molecular proteins; PCL polycaprolactone; PFC perfluorocarbons; PFO pericapsular fibrotic overgrowth; PLL poly-l-lysine; PMCG ploy methylene-co-guanidine; SPIO super paramagnetic iron oxide; TLR toll-like receptor; TLR4 toll-like receptor 4; TNF-alpha tumour necrosis factor alpha; US ultrasound; VEGF vascular endothelial growth factor
1. Introduction
Type 1 diabetes mellitus (T1DM) is an autoimmune disorder resulting from a progresssive destruction of the insulin-producing beta-cells in the pancreas leading to elevated blood glucose levels [1]. The prolonged high blood glucose levels in patients with T1DM can lead to chronic complications such as retinopathy, neuropathy and nephropathy. Currently, it has been estimated that 387 million people have diabetes worldwide and approximately 38.7 million are known to have T1DM. With its onset predominantly during the first two decades of life, it was estimated in 2013 that more than 79,000 children developed T1DM [2]. The current mainstay treatment for T1DM is the daily subcutaneous administration of insulin to regulate blood glucose levels (BGLs). However, the degree of control of BGLs with exogenous insulin delivery is inferior to that achieved by normal pancreatic insulin-producing cells, which secrete insulin directly into the blood stream in a dynamic fashion to maintain BGLs within a physiological range. With exogenous insulin treatment, BGLs can still fluctuate considerably in some people, resulting in symptoms such as sweating and dizziness when the levels are low, and damage to the eyes and kidneys when levels are high for long periods of time [3]. Furthermore, prolonged and recurrent episodes of hypoglycemia can lead to life-threatening "hypoglycemic unawareness syndrome" which accounts for 6-10% of all deaths in T1DM [4]. The limitations of exogenous insulin administration to achieve normal BGL homeostasis have led to the development of alternative therapies, including closed loop pumps, whole organ transplantation, and beta-cell replacement.
Whole pancreas transplantation has been carried out since 1966 and has been demonstrated to routinely provide tight blood glucose control without hypoglycaemic episodes and prevent the progression of diabetes complications [5, 6]. New surgical techniques and newer immunosuppressive strategies have improved the graft survival rate dramatically with a survival rate of >95% at one year and > 83% after 5 years post-transplantation [7]. However, though clinically successful, this procedure has its drawbacks, involving major surgical intervention with associated mortality rates of up to 8% and morbidities such as graft thrombosis, pancreatic fistulae, and pseudocyst formation [8]. Furthermore, the transplanted patients must adhere to a life-long immunosuppressive regime that has potentially serious side effects. As a result, whole pancreas transplantation is most commonly performed in patients undergoing simultaneous kidney transplantation. Some of the limitations of whole pancreas transplantation can be overcome by transplanting only the endocrine component of the pancreas namely the pancreatic islets of Langerhans or 'islets'.
Islet transplantation can be performed as a minimally invasive procedure without many of the associated complications of whole pancreas transplantation. At the same time, it can still provide better glycemic control compared with conventional exogenous insulin treatment. Therefore, potentially, this becomes an attractive option for a wider range of patients with T1DM. Since the landmark study of islet transplantation published in 2000 by the Edmonton group using a steroid-free immunosuppressive protocol [9], several centers and islet consortia around the world have reported promising results with human islet transplantation. Islet graft survival rates improved significantly with most series reporting 55-60% of patients being insulin independent at 1 year. In some selective studies, as many as 92% were insulin-independent a year following islet transplantation, and 44% were still insulin independent at 3 years [10]. Reports of the Collaborative Islet Transplant Registry (CITR) concluded that islet transplantation could achieve near-normal or normal HbA1c levels and could routinely resolve life-threatening hypoglycemia [11]. Despite the dramatic progress made in islet transplantation, longer-term graft function and insulin independence is not achieved routinely. Several non-immunosuppressive and immunosuppressive related factors are associated with the long-term failure of transplanted islets. Factors not associated with immunosuppression may also lead to graft failure, including poor islet quality, insufficient islet mass [12], poor vascularization, relative hypoxia of the transplanted islets leading to graft failure [13], and graft loss by instant blood-mediated inflammatory reaction (IBMIR) [14]. Factors associated with immunosuppression conc ern the immunosuppressive medication that islet recipients receive to prevent allograft rejection. Apart from the clinical side-effects associated with chronic immunosuppression, such as renal dysfunction, increased susceptibility to infection, and increased risk of cancer, immunosuppressive drugs can also have deleterious effects on the transplanted islet themselves which in turn can lead to graft failure. Even the most promising rapamycin-based immunosuppression protocol adopted by the Edmonton group was found to have deleterious effects on both rodent and human islets and to induce beta-cell apoptosis [15]. Other agents such as tacrolimus were found to be more toxic to beta-cells than cyclosporine [16]. Several new immunosuppressive protocols such as T-cell depletion (hOKT3gamma 1 [Ala-Ala-]), B-cell depletion (rituximab) or induction of peripheral tolerance (anti-CD40L and belatacept) have been tested both in preclinical and clinical studies [17]. However, at present there is no single immunosuppression protocol that can prevent islet allograft rejection without being toxic to the grafted islets and without causing serious side-effects to transplant recipients. One strategy to overcome the use of immunosuppressive medications therefore is to place the islets within an immunoisolation device using encapsulation technology.
2. Islet encapsulation
Encapsulation of pancreatic islets involves encasing the pancreatic islets within a protective immunoisolation device to overcome immune mediated destruction of the graft without the need for toxic immunosuppression. Generally, the immunoisolation device is made of a semi-permeable inert material which allows the exchange of glucose, oxygen, nutrients, metabolic waste and insulin, but prevents the entry of large molecules such as immune cells or antibodies. Thus islets encapsulated within an immunoisolation device can also be termed a ‘bioartificial pancreas'. Islet encapsulation can be broadly classified into two categories namely i) macroencapsulation that involves encapsulation of multiple islets within a macro device, and ii) microencapsulation that involves encapsulation of each individual islet.
2.1 Macroencapsulation
Macroencapsulation involves encasing multiple islets within a macrocapsule device (>1 mm). These are classified into two main types, namely intravascular and extravascular. An intravascular macrocapsulation generally involves placing multiple islets within hollow semi-permeable fibers that are then directly connected to the host’s vasculature by vascular anastomoses [18]. Since the device is in direct contact with the blood stream, the islets within the device receive ample oxygen and nutrients thereby promoting islet survival while being protected from the immune system by the fiber membrane. Despite promising animal studies with intravascular devices [19, 20], groups have reported severe problems with embolization and clot formation, thereby preventing the FDA from approving this bioartifical pancreas for clinical trials [21]. Recently, a group from California has developed an intravascular device containing a silicon nanopore membrane to prevent thrombosis by exhibiting greater hydraulic permeability and a superior pore size selectivity [22].
Extravsacular macroencapsulation generally involves places multiple islets within simple diffusion chambers that do not require the creation of intravascular shunts. Extravascular macrodevices are in the form of either tubular or planar diffusion chambers. Tubular diffusion chambers are generally composed of a copolymer of polyacrylonitrile and polyvinylchloride; they have demonstrated good biocompatibility, and provided excellent graft survival in a clinical setting [23]. However, the tubular device was weak structurally and is susceptible to rupture and required large islet numbers due to low islet seeding density [24]. These drawbacks of tubular devices were overcome using planar devices which were more stable and provided a flat configuration thereby enhancing the islet seeding density. Islet Sheet is one example of the planar flat sheet devices designed by Islet Sheet Medical; it has been demonstrated to provide excellent graft survival both in an allogeneic and xenogeneic transplantation setting [25, 26]. However, the Islet Sheet devices are yet to be tested in clinical trials for their safety and efficacy. One of the major advantages of the extravascular macrodevices is that they can be retrieved easily and completely from the recipients if there are any safety concerns. The major disadvantage with these devices is the limited oxygen diffusion leading to hypoxia and central necrosis of the implanted islets. The issue of limited oxygen diffusion was overcome by another macrodevice, the Theracyte, which was designed with an outer membrane that facilitated neovascularization [27]. Islets encapsulated within Theracyte devices have been shown survived for extended period of time both in an allo- and xeno- transplantation models [28, 29]. A modified version of the Theracyte device, namely the Encaptra® Drug Delivery System (EN250 device) designed by Viacyte, is currently being tested for safety in a Phase I/II clinical trial [30]. Another approach to overcome limited oxygen diffusion is to supply the macrodevice with an exogenous source of oxygen. This approach has been adopted by Beta-O2 Technologies Ltd. that has developed an oxygen-refueled macrochamber (βAir). This has generated promising data in preclinical models [31, 32]. A pilot clinical trial carried out with human islets encapsulated in βAir demonstrated preserved islet morphology and persistent graft function up to 6 months post-transplantation though insulin independence was not achieved [33]. Recently, another Phase I clinical trial has been started to continue evaluating the safety and efficacy of implanting the βAir macrodevice into human subjects.
2.2 Microencapsulation
Microencapsulation involves encasing single or a small number of islets within microcapsules, and offers several advantages compared with macroencapsulation. Microcapsules are generally spherical in shape, thereby providing a large surface area/volume ratio, and thus maximizing the transport of oxygen and nutrients essential for islet survival [34]. Further, the microcapsules are mechanically stable and simpler to produce, thereby giving freedom to alter parameters such as capsule size, permeability and thickness. They can be implanted using a minimally invasive procedure and the smooth spherical geometry minimizes foreign body reaction as opposed to host inflammatory reactions seen against rough surfaces [35]. However, the main disadvantage is the difficulty in retrieving the microcapsules from the implanted site. The microcapsules are generally made from polymers that form hydrogels and many natural polymers such as alginate, agarose, chitosan and synthetic polymers such as polyethylene glycol, poly methyl methacrylates, 2-hydroxyethylmethacrylate have been frequently employed [36]. Of these, the naturally occurring alginate polymers are widely used for microencapsulation as they can be produced under physiological conditions without the need for toxic chemicals and without affecting islet viability and function.
Alginate is an anionic polysaccharide obtained from seaweed and comprising monomers of α-L-guluronic acid (G) and its C-5 epimer β-D-mannuronic acid (M) residues joined together by (1-4) glycosidic linkages. Alginates isolated from different species vary widely in their G and M composition as well as the length of each block, and hence affect the physiochemical properties of the alginate microcapsules [37]. The process of preparing microcapsules commonly involves three basic steps namely incorporation of islets within the alginate solution to form a suspension, dispersion of the suspension through an air-driven droplet generator to form alginate droplets and finally gelation of the alginate droplets using divalent cations such as Ca2+ or Ba2+. The binding of these ions is highly selective and is a result of anonic interaction between the carboxyl groups and the cations which strongly depends on the alginate composition [38]. Microcapsules made from high G alginate are stronger, more stable, and swell less compared to those made from high M alginate as the divalent cations bind strongly to G than to M residues. To reduce the permeability and increase the stability of alginate microcapsules, a polycation layer is generally added to the alginate gel core as a second layer followed by an outer layer of alginate. The polycation most commonly used is poly-l-lysine, although other polycations such as poly-l-ornithine have also been employed. But the most commonly used microencapsulation system for islet encapsulation employed either alginate-poly-l-lysine-alginate (APA) or barium alginate microcapsules.
2.3 Preclinical studies with microcapsules
The first animal study using encapsulated islets was conducted by Lim and Sun in 1980. These authors showed that encapsulated islets transplanted intraperitoneally into diabetic rats could maintain normoglycemia for up to 3 weeks in contrast to non-encaspulated islets which survived only for a week when transplanted beneath the renal capsule [39]. In that study, they used APA microcapsules made from high M alginate which had a liquid capsule core. However, high M alginate is associated with increased swelling. Therefore, APA microcapsules with a liquid core were unstable. Based on this finding, many studies were performed to replace the liquid core with a solid core and high G alginate was utilized as they are less susceptible to swelling and produced stable and mechanically stronger microcapsules. A study by de Vos et al. demonstrated that high M alginate led to insufficient islet encapsulation and islet protrusion [40]. Furthermore, transplantation of high M APA microcapsules stimulated macrophages and lymphocytes leading to PFO and high M alginate has been shown to be more immuonogeneic than high G alginate [41, 42]. However, a consensus has not been reached within the scientific community regarding the biocompatibility of high G or M alginates. Despite this, many animal studies have been carried out using islets encapsulated within APA microcapsules and transplanted into rodents, dogs and monkeys [43]. Long term survival of both allogeneic and xenogeneic islets encapsulated in APA microcapsules without immunosuppression has been reported [43]. A slightly modified microcapsule containing poly-l-ornithine instead of PLL provided better graft survival of pig islets when xenotransplanted into cynomolgus monkeys [44]. Despite these encouraging results, APA microcapsules suffer from a major shortcoming. The polycationic PLL coating degrades over time and are found to be highly immunogenic which makes the APA microcapsules bio-incompatible and unstable in the long run [45, 46].
To overcome the problems associated with PLL in APA microcapsules, the alginate was cross-linked directly with barium without the addition of PLL to form barium alginate microcapsules. It has been demonstrated that cross-linking high G alginate with Ba2+ ions resulted in microcapsules of higher strength and stability and that the resulting microcapsules were less permeable to IgG compared to other microcapsules [47]. Further, the absence of PLL makes these barium alginate microcapsules more biocompatible compared to APA microcapsules [48]. Many animal studies have been carried out both by our group [49-51], and by others [43, 52], which have demonstrated the capability of these barium alginate microcapsules to provide long-term immunoprotection both in an allo- and xenotransplantation setting. However, even in the absence of the immunogeneic PLL, the barium alginate microcapsules were susceptible to PFO. Whether the inflammatory response seen as PFO is directed against the encapsulated tissue or the alginate material is poorly understood. This is due to widely varying results, with outcomes influenced by varied factors such as the alginate purity and composition, polycations used and the animal models employed. Indeed, we have previsouly shown that the PFO directed against alginate microcapsules is species specific with barium alginate microcapsules being devoid of PFO when tested in small animals like rodents but elicited a strong PFO when transplanted into a large animal such as baboon [53].
Furthermore, it has been reported previously that empty microcapsules made from a purified source of alginate do not develop PFO when implanted into the peritoneal cavity of rodents [54, 55], but do provoke extensive PFO when implanted into the portal vein of pigs [56]. To our knowledge, there are no studies reported in the literature assessing the biocompatibility of empty barium alginate microcapsules when implanted into the peritoneal cavity or omentum of a large animal model. Hence we carried out a study in pigs (Figure 1) to test the biocompatibility of empty barium alginate microcapsules transplanted either intraperitoneally (n = 2) or into the omental pouch (n = 2). For intraperitoneal transplants, the pigs were transplanted with ~100,000 microcapsules, which they were retrieved at 1 week, 3 weeks, 8 weeks, and 1 year post-transplantation. Laparoscopic examination of the peritoneal cavity at 1 and 3 weeks demonstrated free microcapsules that could be flushed out by saline, and microcapsules were seen both singly and in clumps (Figures 2A and 2B). However, at 8 weeks post-transplantation, the microcapsules were found adherent to abdominal structures with no evidence of free-floating microcapsules which can be flushed out easily (Figure 2C). At 1 year post-transplantation, no free microcapsules could be found within the peritoneal cavity and papules, consistent with clusters of adherent microcapsules, were visible at a number of sites including the posterior peritoneal wall (Figure 2D), omentum (Figure 2E), and liver (Figure 2F). For the omental pouch experiments, the pigs were transplanted with ~37,000 microcapsules and retrieved at 8 weeks post-transplantation. Analysis of the retrieved graft showed that microcapsules were not free floating and were found embedded in the omental tissue.
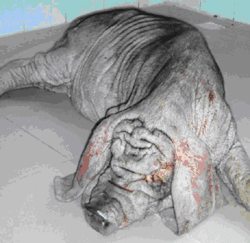 |
 |
Figure 1. Biocompatibility testing of barium alginate microcapsules in pigs. Empty barium alginate microcapsules were transplanted into the peritoneal cavity and omental pouch of Erhualian pigs and the host immune response assessed at varied time points post-transplantation. |
|
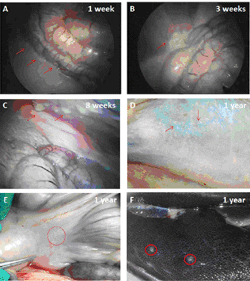 |
 |
Figure 2. Graft retrieval from the peritoneal cavity of pigs. Laparoscopy images show the presence of large number of free transparent microcapsules at week 1 (A) and week 3 (B) respectively. At week 8 the number of free microcapsules was less and most capsules were found adhering to abdominal structures (C). No free microcapsules were seen by 1 year post-transplantation and papules, consistent with clusters of adherent microcapsules, were visible at a number of sites including posterior peritoneal wall (D), omentum (E) and liver (F). |
|
Histology of the grafts retrieved at 8 weeks post-transplantation demonstrated that capsules were scattered throughout the pouch and lay adjacent to each other mostly surrounded by a thin layer of fibrous tissue which was well vascularized (Figures 3A and 3B). A cellular response was observed around some capsules and in cases a visible cellular infiltrate was seen (Figures 3C and 3D). This study demonstrated that the barium alginate microcapsules are not biocompatible in the pig model, at least when placed in the peritoneal cavity of the pig, and elicit a foreign body response depicted as PFO when transplanted both intraperitoneal and into the omental pouch. The thin layer of well vascularized fibrous tissue around the microcapsules in the omentum offers some hope that if insulin-producing cells were placed in microcapsules transplanted at this site, they might survive and function.
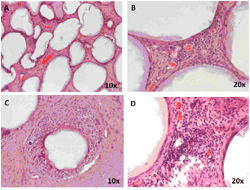 |
 |
Figure 3. Graft retrieval from the omental pouch at 8 weeks post-transplantation. Multiple capsules were located adjacent to each other (A), most surrounded by a thin layer of fibrous tissue, which was well vascularised (B). A cellular response was observed around some capsules (C) and in a small number of cases, there is a visible cellular infiltrate (D). |
|
2.4 Clinical studies with microcapsles
The first human study of encapsulated islets was carried out in 1994 by Soon Shiong et al. on a diabetic patient who was on immunosuppressive drugs for a functioning kidney allograft [57]. The diabetic patient received an initial intraperitoneal infusion of islets (10,000 IEQ/kg) encapsulated within APA microcapsules followed by an intermediary infusion of encapsulated islets (5000 IEQ/kg) 6 months after the first infusion. Tight glycemic control was achieved and the patient remained insulin independent for 9 months post-transplantation. Since then many clinical trials have been carried out using encapsulated islets. However, none of these were able to achieve insulin independence, as reported in the study Soon Shiong et al.
Almost 12 years later, Calafiore et al. carried out a clinical trial with human islets encapsulated within alginate-PLO microcapsules without immunosuppression [58, 59]. In that study, four diabetic patients were implanted intraperitoneally with encapsulated islets ranging from 5000-15,000 IEQ/kg (under local anesthesia and ultrasound guidance). All the transplanted patients tested positive for serum C-peptide response a marker for islet graft function throughout 3 years of post-transplant follow-up. The study also reported the need for a reduction in the exogenous insulin requirement in all the patients, with transient insulin independence in one patient. There was no induction of HLA class I or II antibodies and all the patients tested negative for anti-GAD65 antibodies. However, 7 years post-transplant all patients became insulin-dependent again, and were back to their original exogenous insulin requirements prior to transplantation. Microcapsule retrieval at 5 years post-transplant from a patient with abdominal discomfort demonstrated a cyst formation with fibrotic lump that contained mostly intact microcapsules with no viable islets. The authors reported the cyst formation to be attributed to surgical error as the microcapsules were inadvertently placed beneath the muscle fascia instead of the intraperitoneal cavity.
Between 2005 and 2006, two companies, namely Amycte, Inc., and Novocell, Inc. (now ViaCyte, Inc.), planned to carry out clinical trials with encapsulated islets in type 1 diabetic patients. Amycte, Inc. planned to encapsulate human islets with APA microcapsules, which were then embedded into a macrodevice before implantation into twelve type 1 diabetic patients. Novocell, Inc. started a phase1/2 clinical trial, employing PEG-encapsulated islet allografts; twelve patients were enrolled in this trial and received subcutaneous implants. However, the study was terminated by the company after only limited efficacy was observed in the first two recipients [21]. Currently, there is not much information available about these clinical trials.
In 2007, Living Cell Technologies (LCT) reported on the outcome of a clinical trial initiated in 1996 involving encapsulated porcine islet transplantation [60]. In that trial a 41-year old Caucasian male with type 1 diabetes received an intraperitoneal transplant of porcine islets (15,000 IEQ/kg) encapsulated within alginate-PLO microcapsules. By 3 months post-transplant, his exogenous insulin requirements reduced significantly by 30% and had improved glycemic control as reflected in the reduction of the total glycated hemoglobin. Moreover, urinary C-peptide levels peaked at 4 months and remained detectable at 11 months. However, by 11 months post-transplant the graft became non-functional and the exogenous insulin dose returned to the pre-transplant levels. The patient was monitored long-term thereafter and showed no evidence of porcine viral or retroviral infection with a laparoscopy being carried out 9.5 years post-transplant. Laparoscopy revealed opaque nodules spread throughout the omentum and mesentery, and more importantly, no signs of peritoneal reaction or fibrosis. Biopsies of the nodules demonstrated opaque microcapsules that were intact with live cell clusters that stained sparsely for insulin and glucagon and produced a small amount of insulin in response to an in vitro glucose challenge. Recently, LCT reported on a phase 1/2 clinical trial of DIABECELL conducted in New Zealand and Russia enrolling fourteen type 1 diabetic patients [61]. Reports from the company website state that the first four patients transplanted with encapsulated porcine islets (10,000 IEQ/kg) had a 76% reduction in hypoglycemic unawareness episodes after 7-12 months post-transplantation follow-up. Another eight patients were transplanted with 15,000 IEQ/kg (four patients) and 20,000 IEQ/kg (four patients) of encapsulated porcine islets, and are currently being followed up. The last two patients were transplanted with 5000 IEQ/kg of encapsulated islets and were enrolled to construct the dose ranging data required for a phase 3 clinical trial. There has been a lowering of the level of HbA1c to <7% for more than 600 days post transplantation, with a significant reduction of unrecognized hypoglycemic events. Recently, the company website reported that a registration study has been launched in 2013 for a phase 2b/3 trial, and DIABECELL is expected to be available in the market by 2016 [62].
We also carried out a phase 1 clinical trial ('The Seaweed Diabetes Trial') with encapsulated human islets encapsulated within barium alginate microcapsules without any polycations such as PLL or PLO [63]. Four type 1 diabetic patients with no detectable C-peptide received an intraperitoneal infusion of barium alginate encapsulated islets with a mean of 178,000 IEQ per infusion. One patient received four islet infusions over 7 months and another received two infusions 10 months apart, and the remaining two recipients received one infusion each. No immunosuppression was used, but the recipients received a mild anti-inflammatory agent and antioxidants. Urinary C-peptide was detected in all the patients on day 1 post transplantation, and the blood glucose levels and insulin requirements decreased to 36 ± 8% and 22 ± 3%, respectively, but not thereafter. Urinary C-peptide became undetectable at 1-4 weeks in 3 of the recipients. In the one patient receiving multiple infusions, urinary C-peptide was detected at 6 weeks and remained detectable by 2.5 years post-transplantation. However, the small amount of insulin produced in that patient altered neither the exogenous insulin requirements nor the glycemic control. In contrast to other clinical studies discussed above, antibodies against GAD were detected in three patients and remained detectable 1.1-2.5 years after the first infusion. Cytotoxic antibodies were detected in two patients and remained detectable at 0.6-1.9 years after the first infusion. To better understand what was happening to the encapsulated islets, a laparoscopy was carried out on the multi-islet recipient at 16 months post-transplantation. Large number of microcapsules were found scattered throughout the peritoneal cavity, and were found attached to the parietal peritoneum, spleen, omentum, and kidney. A biopsy demonstrated that the microcapsules remained intact, and were surrounded by layers of fibrous tissue with necrotic islets. Although a clinical benefit could not be demonstrated, this study showed that allografting of barium alginate encapsulated human islets is safe with no major side-effects or infection to the transplanted recipients.
Mostly recently, Jacobs-Tulleneers-Thevissen et al. has published on a human trial employing Ca2+/Ba2+ alginate microbeads containing allogenic islets [64]. Encapsulated human islets (300,000 IEQ) were injected into the peritoneal cavity of a 61-year old female type 1 diabetic patient under maintenance immunosuppression for an intraportal islet transplantation received 5 years earlier. Plasma C-peptide levels increased above the pre-transplant levels in the first 12 weeks but reached a threshold within the first week. However, there was no reduction in the exogenous insulin requirements and diabetes auto-antibody status remained unchanged with no induction of cytotoxic antibodies. A laparoscopy carried out a 3 month post-transplantation, reported single as well as cluster of microcapsules spread throughout the peritoneal cavity. The numerous single microcapsules loosely attached to the peritoneum were easily retrievable, free of fibrosis and marginally functional which the authors relate to poor viability of the retrieved islets. However, the majority of the microcapsules were seen clustered at several sites including the greater omentum and pelvic floor and were surrounded by vascularized fibrous tissue infiltrated with immune cells and mostly contained debris.
From the above clinical studies, it can been concluded that both alginate-polycation-alginate and Ca2+/Ba2+ alginate microcapsules were employed for encapsulation, and both were transplanted in diabetic recipients at the same site, namely the peritoneal cavity. In all the cases, small amounts of C-peptide were detectable at different time points post-transplantation, suggesting microcapsules to offer some degree of immune protection to islets, at least in the early stages. However, in the long term, microencapsulated islet grafts eventually failed, and offered no clinical benefit to the diabetic recipients, although no adverse reactions were reported. The reasons for graft failure are not clear, and are attributed to many factors such as quality of the isolated islets, graft volume, and encapsulated islet viability, all of which can influence the transplant outcome. However, laparoscopic retrieval of microcapsules carried out in some clinical studies suggested that clumping of microcapsules and formation of PFO are major factors resulting in loss of graft function [63, 64]. Whether the PFO is directed against the microcapsules or the encapsulated tissue remains largely unclear, the contribution of alginate material to PFO cannot be ruled out as antibodies towards alginate were detected as early as 20 days post-transplantation, though the levels declined thereafter [65]. However, since empty microcapsules have not been tested in humans, their contribution to PFO remains unanswered. Despite this, and based on the available information, it can be said that PFO is one of the major causes for the failure of encapsulated islets in a clinical setting.
3. Strategies to prevent/reduce PFO and improve encapsulated islet survival
The ideal microencapsulation device should have the following characteristics:
- It should be completely inert so as not to induce an immunological or fibrotic reaction
- It should be robust without disintegration (i.e., non-biodegradable) over prolonged periods of time
- It should have a smooth topography without rough surface
- It should be compatible both with the encapsulated cells and the host to be bio-tolerable and not induce an immunological and fibrotic reaction
The alginate hydrogels used in encapsulation largely fulfill the above characteristics in being inert, robust, having a smooth surface, and being hydrophilic, which highly reduces protein adsorption and cell attachment. Microencapsulated islets have also been shown to normalize glucose levels for extended periods in both preclinical models of allo- and xenotransplantation [66]. However, in all these studies, graft survival was limited, and varied considerably, ranging from months to a few years. Furthermore, phase 1 clinical studies with encapsulated islets resulted in poor outcomes, with PFO being the major factor responsible for graft failure [67-69]. The factors contributing to PFO are poorly understood, and are often seen as a foreign body reaction to the alginate microcapsules or host inflammatory response to antigens shed by encapsulated tissue [70, 71]. Thus, combating PFO is crucial for improving transplant outcomes with encapsulated islets, and is actively being pursued by varied research groups. Below we discuss the various strategies pursued or being pursued to reduce/prevent PFO and enhance graft survival (as summarized in Table 1, see Appendix).
3.1 Alginate purification
Alginate purity is one of the major factors affecting the biocompatibility of alginate-based microcapsules leading to fibrotic overgrowth. Alginates are obtained from natural sources and are known to contain immunogenic contaminants such as proteins, polyphenols, and endotoxins [72]. Endotoxins can stimulate the immune system while polyphenols and proteins can be toxic to the encapsulated cells. Lipopolysaccharide (LPS) is the most common endotoxin found in alginate polymers and can bind to toll-like receptor 4 (TLR4) to induce an inflammatory response in a wide variety of immune cells [73]. Studies have demonstrated that impurities in alginate are a key culprit, contributing to poor biocompatibility, and consequently leading to poor graft survival due to PFO [74-76]. The majority of studies used alginate which has been sourced commercially and marketed as being 'highly purified', despite the alginate microcapsules made from them being immunogenic. Microcapsules made from these alginate sources have been shown to activate the innate immune system and induce the release of proinflammatory cytokines IL-1β, TNF-α, and IL-6 from both murine and human monocytes and macrophages [77-79]. A recent study used a cell based assay as a screening tool to identify impurities and found that commercially sourced alginate labeled as "ultra-pure" still contain impurities such as peptidoglycan and lipoteichoic acid which are referred to as pathogen-associated molecular patterns (PAMPs) [80]. The study also elegantly demonstrated that the PAMPs can act as ligands for toll-like receptors (TLR) and pattern recognition receptors (PRRs), thereby activating NF-kB resulting in activation of the innate immune system and release of proinflammatory cytokines. Most of these cytokines are small enough to permeate through the microcapsule pores, and have deleterious effects on encapsulated islet cells [81, 82]. Upon transplantation into immunocompetent rats, these unpurified alginate with PAMPs elicited a PFO and poor biocompatibility. On the other hand, purified alginate with low levels of PAMPs demonstrated superior biocompatibility with no PFO upon transplantation [83]. However, removal of all these impurities is a cumbersome process and as such all purification procedures focused only on endotoxin LPS. Therefore, novel screening tools and purification procedures need to be developed to detect and remove other PAMPs such as peptidoglycan and lipoteichoic acid. A recent study suggested the development of a cell based screening assay to identify PAMPs in alginate polymers [80]. This clearly demonstrates the need for purifying alginate before being used for encapsulation and transplantation purposes. However, many groups have difficulties in consistently producing ultra-pure alginates, despite using the same purification procedures, which leads to considerable inter-lab variations [84, 85]. This problem highlights the crucial need for the optimization of the purification procedure, and development of a standard operating procedure to purify alginate employed in islet encapsulation.
3.2 Alginate composition and geometry
Apart from purity, alginate composition also plays a major role in determining the biocompatibility. Alginate is a polysaccharide and is made of repeating units of guluronic (G) and mannuronic (M) acid, and the ratio of G/M greatly affects the physiochemical properties and play a major role in determining the biocompatibility of alginate microcapsules. Alginate microcapsules made from high-G alginate are more stable compared with high-M, whereas microcapsules from high-M alginate can provide selective permeability to immunoglobulins and immune cells, thereby providing better immunoprotection [86, 87]. However, some studies have reported alginate microcapsules with high M to be more immunogenic leading to PFO [88, 89], whereas others have reported the oppotite [90, 91]. Another study demonstrated that microcapsules made from intermediate-G alginate were biocompatible and free of PFO compared to microcapsules made from high-G alginate [92].
Apart from G/M ratio, alginate viscosity and molecular weight also plays a major role in determining the biocompatibility of alginate microcapsules. It has been demonstrated that microcapsules prepared from low, but not medium, viscosity alginate elicited a strong PFO, stressing the need to remove low molecular weight fractions during purification procedure to enhance biocompatibility [93]. Another study demonstrated that alginate implants made from high viscosity and high mannuronic acid, but without guluronic acid, demonstrated better stability, no PFO, and improved graft survival in a xenotransplantation setting [94].
Another factor that plays a critical role in determining biocompatibility is capsule geometry. Traditional microcapsules comprise fixed-diameter spheres ranging from 700-1500 µm, and have been reported to have large diffusion barriers leading to delayed glucose sensing and insulin responsiveness and poor oxygenation, resulting in hypoxia and necrosis of the encapsulated islets, thereby triggering the immune response leading to PFO [95]. These drawbacks could be overcome using smaller microcapsules, and studies have demonstrated that smaller microcapsules can provide better insulin kinetics and improved cell oxygenation [96, 97]. Furthermore, smaller microcapsules of the order of 250-350 μm are found to be biocompatible with less PFO compared with the traditional microcapsules [98, 99]. However, a recent study elegantly suggested another strategiy by showing that larger alginate microcapsules of 1.5 mm diameter have better biocompatibility, and significantly mitigated PFO compared with smaller 0.5 mm capsules when transplanted both into C57BL/6 and in non-human primates [100]. The authors also demonstrated that islets encapsulated within these larger 1.5 mm capsules remained viable, had similar insulin kinetics, and provided better glycemic control in a xenotransplantation setting with significantly less PFO compared with smaller microcapsules. In conclusion, it can be stated that not all results are in agreement. There is still a need for a better understanding of the alginate composition and microcapsule geometry in influencing alginate microcapsule biocompatibility.
3.3 Modification of alginate microcapsules
Traditionally, alginate microcapsules used for islet encapsulation are made of alginate/poly-l-lysine-alginate complex where ploy-l-lysine (PLL) has been added to reduce porosity and osmotic swelling [101]. However, several studies have shown that the bound PLL is immunogeneic and increases the host reaction resulting in PFO and being detrimental to the encapsulated islets [102, 103]. Over the years, many polycations such as poly-L-ornithine [104], chitosan [105], poly-d-lysine [106], and diblock copolymers [107] have been tried as an alternative to PLL to reduce PFO and improve biocompatibility. Some studies have investigated the incorporation of anti-inflammatory agents into alginate microcapsules to combat PFO. Hybrid curcumin-alginate microcapsules significantly reduced PFO and improved glycemic control when encapsulated rat islets were xenotransplanted into immunocompetent mice [108]. Similarly, composite alginate microcapsules containing ketoprofen loaded biodegradable microspheres demonstrated better biocompatibility with no PFO compared to unmodified microcapsules when transplanted into the peritoneal cavity of CD-1 mice [109]. Alginate microcapsules co-encapsulated with steroids (namely dexamethasone) demonstrated better biocompatibility and were 'free floating' with no PFO when retrieved from the peritoneal cavity at 4 weeks post-transplantation [110]. Another study demonstrated that loading anti-inflammatory drug pentoxifylline into the inner layer of dextran-spermine coated alginate microcapsules had enhanced immunosuppressive effects in vitro when encapsulated islets were co-cultured with lymphocytes [111]. A recent study demonstrated the usefulness of incorporating ursodeoxycholic acid into the alginate matrix which produced microcapsules of good stability and cell viability [112, 113]. Another study demonstrated that co-encapsulation of islets with HMGB1 A box protein significantly attenuated TNF-a secretion when co-cultured with macrophages and improved graft survival by 2 fold in a xenotransplantation setting [114].
Another approach to improve biocompatibility and reduce PFO is by modifying the surface chemistry of alginate microacspules. One study demonstrated that coating alginate-chitosan microbeads with methoxy poly (ethylene glycol) (MPEG) created a more biocompatible and protein repellent surface which prevented gamma globulin (IgG) and fibrinogen protein adsorption important in PFO formation [115]. Another study showed that genipin-modified empty alginate/PLO/alginate microbeads exhibited improved hydrophilicity and biocompatibility with less PFO when transplanted into the peritoneal cavity of immunocompetent mice [116]. However, the beneficial effect was lost when genipin modified microcapsules containing islets were xenotransplanted. The same group recently demonstrated that coating photocross-linkable methacrylated glycol chitosan on alginate microcapsules improved capsule properties without affecting cell viability and function in vitro, and demonstrated good biocompatibility in vivo with reduced PFO when compared to alginate/PLO/alginate microcapsules [117]. Coating alginate microcapsules with poly (methylene-co-guanidine) (PMCG) resulted in stronger microcapsules with increased stability compared to alginate/PLL/alginate capsules. However, its in vivo biocompatibility is yet to be tested [118]. A recent study demonstrated that coating alginate microcapsules with rapamycin-containing polyethylene glycol decreased the proliferation of macrophage cells and significantly reduced PFO compared to non-coated alginate microcapsules [119]. In yet another study, it has been shown that alginate-PEG microcapsules, when transplanted, improved biocompatibility and engraftment of allogenic islets only at the site of the peritoneal cavity, but not the highly vascularized epididymal fat pad in a rodent model [120]. This study suggests that transplant site also affect graft outcomes in addition to microcapsule composition.
We recently showed that modifying the surface of alginate microcapsules with a patented macromolecular heparin conjugate improved biocompatibility and significantly reduced PFO in both syngeneic and allogeneic transplantation models with no benefit seen in a xenogeneic model [121]. However, another study concluded that coating alginate microcapsules with a chemokine CXCL12 improved biocompatibility and resulted in long term allo- and xeno- islet survival and function by recruiting the immunosuppressive regulatory T cells to the transplant site [122]. Recently, we have demonstrated that coating the surface of alginate microcapsules with zwitterionic block co-polymers significantly reduced PFO, and improved graft survival in a xenotransplantation setting (unpublished data). A recent elegant study showed that chemical modification of alginate using triazole-thiomorpholine dioxide resisted PFO when empty alginate microspheres were implanted into both rodents and non-human primates [123], and stem cell-derived β-cells encapsulated within these alginate microspheres provided long-term glycemic control without immunosuppression in a xenotransplantation setting [124]. Another recent study has shown that coating alginate microcapsules with chitosan significantly improved its biocompatibility by reducing PFO. In this study, the authors showed that chitosan-coated alginate microcapsules significantly reduced PFO and normalized blood glucose levels for up to 1 year both in a canine allotransplantation and rodent xenotransplantation model [125]. However, despite all the surface-modification strategies to reduce PFO and improve graft survival in small animal models, as outlined above, none of them have been successful in large animals, thereby undermining the clinical significance of the above approaches. Therefore, a concentrated research effort is needed to develop novel biocompatible alginate microcapsules which are free of PFO and which can improve graft survival both in an allo- and xeno-transplant setting. Clinical trials should test the most promising microcapsules, containing human insulin-producing cells, in normalizing blood sugar levels of recipient humans without immunosuppression.
3.4 Co-encapsulation with companion cells
Another strategy to reduce PFO and enhance encapsulated islet survival is by co-encapsulating with immunomodulatory companion or bioengineered cells. Sertoli cells have been widely studied as an immunomodulatory companion cell, and have been shown to inhibit T- and B-cell proliferation and IL-12 production [126]. Co-transplantation of non-encapsulated islets with Sertoli cells has been shown to be beneficial in enhancing graft survival in allo- [127], xeno- [128], and autoimmune transplant models [129]. Further, studies have found that co-encapsulation of islets with Sertoli cells improved graft survival and function in a xenotransplantation setting by producing local immunosuppressive factors [130, 131]. However, the effect of Sertoli cell co-encapsulation on PFO was not reported in those studies. Other attractive companion cells, which are widely investigated for their immunomodulatory properties, are mesenchymal stem cells (MSC). MSC inhibit immune responses by releasing soluble cytokines and growth factors to neighbouring cells, resulting in a localized immunosuppressive effect [132, 133]. The immunomodulatory properties of MSC have been widely exploited, and MSC have been used in several studies to enhance islet survival and improve transplantation outcomes [134-136]. A recent study highlighted the benefit of co-encapsulating islets with MSC to enhance insulin secretion and improve transplantation outcomes in a syngeneic setting with no benefit seen in PFO reduction [137]. In a macroencapsulation study, MSC co-encapsulation with pig islets improved graft survival and function by enhancing oxygenation and neoangiogenesis in subcutaneous transplants with no apparent positive MSC effects on the occurrence of PFO [138]. As there are no studies examining the direct effects of MSC co-encapsulation on PFO and graft survival, we recently investigated this strategy to reduce PFO in different transplantation setting. We found that co-encapsulating MSC significantly reduced PFO and improved graft survival both in allotransplantation (unpublished data) and xenotransplantation models (unpublished data). Furthermore, we also found that activating MSC prior to transplantation significantly enhanced their immunosuppressive properties and co-encapsulation of activated MSC significantly reduced PFO, improved graft survival, and provided better glycemic control in an allotransplantation setting (unpublished data). Apart from Sertoli cells and MSC, genetically modified cells have also been co-encapsulated to enhance islet survival. Co-encapsulation of islets with bioengineered IGF-II producing TM4 cells improved beta-cell survival, and provided better glycemic control. However, the effect on PFO was not reported in that study [139].
3.5 Administration of pharmacological agents
Another strategy which has been applied to prevent or reduce PFO is the use of immunosuppressive drugs. It has been demonstrated that short-term immunosuppression for 10 days with either rapamycin, tacrolimus, combination of rapamycin and tacrolimus, or gadolinium-chloride did not prevent PFO, but reduce its thickness around the intraportally implanted empty microcapsules [140]. Another study demonstrated that continuous subcutaneous delivery of the immunosuppressive drug 15-deoxyspergualin for 4 weeks had a dose-dependent effect on reducing PFO and prolonging graft survival when xenotransplanted into BalB/c mice [141]. Oral administration of an anti-fibrotic agent, HOE 077, reduced PFO and improved encapsulated porcine islet survival when xenotransplanted into an immunocompetent mouse [142]. A research group in Chicago has shown the benefit of using a 2-week long T-cell-directed immunosuppressive medication and TNF-a blocker to prevent PFO [143]. They demonstrated that the immunosuppressive regime could prevent PFO on empty Ca2+/Ba2+ alginate microcapsules transplanted into the peritoneal cavity of cynomologous monkeys only for the time period when the medications were administered. The above studies require the extended use of immunosuppressants to prevent PFO and improve graft survival. However, this defeats the goal of the encapsulation strategy, and is not being pursued by many research groups.
3.6 Transplant site
The choice of the anatomical transplant site is based on where the host's immune system might have minimal impact on the transplanted material, so called 'immune privilege'. An ideal transplant site should have features like low immune exposure, easy retrievability of implanted capsules, access to the host's vasculature with a potential for the implanted graft to neovascularize, and adequate space to accommodate the desired quantity of implanted microcapsules [144]. Non-encapsulated islets are generally transplanted into the liver via the portal vein, as this is a highly vascularized site enabling the transplanted islets to receive sufficient oxygen. However, infusion into the portal vein is not possible with microencapsulated islets due to the large graft volume, and therefore, microencapsulated islets are generally transplanted into the peritoneal cavity. Poor revascularization, high immunogenicity, and difficulty in retrieval of the transplanted capsules make the peritoneal cavity an unfavorable transplant site for microencapsulated islets. Moreover, the influence of gravity on the placement of the microcapsules may cause them to fall and clump together within the pelvic cavity in bipedal hosts, which is another major disadvantage. Furthermore, microcapsules prevent the revascularization process, and microencapsulated islets are subjected to chronic hypoxic stress, which hampers their ability to function properly. This explains the requirement for large numbers of encapsulated islets to normalize glucose levels compared to non-encapsulated islets [145]. Microencapsulated islets subjected to hypoxic stress are known to secrete higher levels of HMGB1, which can trigger an inflammatory response and contribute to PFO [146].
A surgically created omental pouch can help overcome this problem by providing the required space for the large volume of encapsulated islets, as well as enabling close proximity to the vasculature, and hence exerting less hypoxic stress on encapsulated islets. It also ensures easy retrievability of the microcapsules and delivery of insulin into the portal circulation. Studies have demonstrated the benefit of transplanting encapsulated islets into an omental pouch, resulting in long term normoglycemia in rodent models of diabetes using this approach [147]. However, rodent studies have yet to be translated to large animal models, something that is important given that we see a strong PFO when empty barium alginate microcapsules were transplanted into the omental pouch of pigs (Figure 3) in our study. Another transplantation site that has been investigated is the kidney capsule. A large animal study demonstrated that porcine C-peptide was detectable 60 days post-transplantation in two of the seven cynomolgus monkeys when microencapsulated porcine islets were xenotransplanted under the kidney capsule [148]. Although the kidney capsule is highly vascularised, space is limited for the large graft volume associated with encapsulated islets. Other anatomical transplant sites, such as the gastric submucosal space [149], lymph node [150], skeletal muscle [151], pancreas [152], spleen [153], bone marrow [154], the testis, thymus, and anterior eye chamber [155] have all been attempted with non-encapsulated islets, but none of these have been explored for encapsulated islets, mainly because of the space restriction for the large transplant volume.
Another alternative site, which has been widely explored for encapsulated islet transplantation, is the subcutaneous space. The subcutaneous site offers a large surface area, is easy accessible, and permits minimal invasiveness for both transplantation procedure and graft retrieval. However, results from the subcutaneous site transplants have not been encouraging because of poor oxygen tension and slow revascularization, which may compromise islet function and engraftment [155]. A possible explanation might be that the inadequate oxygen supply in the subcutaneous site might have reduced the capacity of encapsulated islets to secrete insulin because they are known to be highly metabolic [155]. Despite poor oxygen supply, subcutaneous transplant site might still be attractive as they are less immunogeneic than the peritoneal cavity. Our own studies have demonstrated that xenotransplantation of encapsulated cells into the peritoneal cavity of an aggressive C57BL/6 mouse results in strong PFO by 3 weeks post-transplantation. However, when encapsulated cells were xenotransplanted subcutaneously the PFO was reduced significantly, despite the capsules being contained within a fibrotic pouch [156]. Thus, transplantation of microencapsulated islets at the subcutaneous site can be adopted as a strategy to reduce PFO and improve islet survival if the issue of poor oxygen supply can be resolved at this site. Several approaches have been attempted to enhance oxygenation at the subcutaneous site, including generating oxygen close to the microcapsules by means of photosynthesis [157] or electrochemical generator [158]. Unfortunately, these oxygen-generating systems cannot produce enough oxygen required in a clinical setting. Other approaches to enhance oxygenation at the subcutaneous site include delivering oxygen through an external source [159], making the islet cells hypoxic resistant [160-162], and inducing neoangiogenesis around the transplanted device. Several groups have demonstrated the benefit of inducing neovascularization using fibroblast growth factor (FGF) [163-165] and hemoglobin cross-linking [166] with improved transplant outcomes in rodents. Other studies have shown that conjugating VEGF to the alginate scaffolds promoted angiogenesis, and improved islet engraftment and function [167, 168]. Recently, an elegant study demonstrated the benefit of using a pre-vascularized subcutaneous device-less site, which provided long term syngeneic, but not allograft, survival (using immunosuppression) by enhancing neovascularization and creating a localized immune tolerance at the transplant site [169].
4. Nanoencapsulation
Another approach to enhancing oxygenation and improving graft survival is to reduce the microcapsule size to decrease the diffusion distance for the oxygen to reach the islet core from the capsule surface. This can be achieved using recent techniques such as conformal coating or layer-by-layer approach. Furthermore, reducing the microcapsule size will significantly reduce the transplant volume, thereby allowing the transplantation of these encapsulated islets into the highly vascularized site such as liver through portal vein infusion.
4.1 Conformal coating
The most commonly used polymer for conformal coating is polyethylene glycol (PEG), which can be cross-linked easily by exposing to UV/Visible light and polymerized in the presence of photo-initiators, thereby forming a nanocapsule around the islets [170]. The first study with conformal coating was carried out by Neocrin Inc., and showed that conformal coated porcine islets normalized blood sugar levels when xenotransplanted into non-immunosuppressed diabetic rodents [171]. However, similar success was not achieved in larger animals as there was a strong immune response against the PEG coatings resulting in graft failure. Another company, Novocell (now ViaCyte), modified the PEG component to improve binding to photo-initiators and to accelerate cross-linking in order to be non-reactive in large animals. The company carried out a pre-clinical nonhuman primate study by subcutaneously implanting conformal coated islets into five diabetic baboons, and demonstrated allograft function in presence of low-dose immunosuppression [172]. Graft retrieval at 20 months post-transplantation showed minimal inflammatory reaction to conformal coated islets. Encouraged by these results, Novocell carried out a phase I/II clinical trial with two type 1 diabetic recipients receiving conformal coated islets transplanted subcutaneously both in the abdomen and back. Although the recipients experienced a decrease in the number of hypoglycemic episodes, both patients did not achieve insulin independence, and the C-peptide levels were lower than expected [172].
A group at the University of Miami developed a new microfluidic method for conformal coating that allowed complete encapsulation of islets within a thin continuous layer of PEG-alginate hydrogel. The group demonstrated that the function of conformally coated islets was not affected both in vitro and in vivo in a syngeneic rodent transplantation model [173]. A recent study demonstrated that allogeneic transplantation of conformally PEG coated islets along with a short-course immunotherapy (anti-LFA-1 antibody) provided a synergistic effect by reversing hyperglycemia and maintaining normoglycemia for more than 100 days with no foreign body reaction [174]. Despite the promising results, PEG is less biocompatible than other hydrogels currently being tested for islet encapsulation, and PEG encapsulated islets are susceptible to cytokine attack [175], which necessitates further investigation before this technology may be applied widely.
4.2 Layer-by-layer coating
In this coating technology, islets are encapsulated by altering positively and negatively charged polymers over the surface of cell clusters, thereby significantly reducing the capsule thickness, improving insulin release kinetics, and promoting diffusion of metabolites and waste products [176]. In 2006, Krol et al. demonstrated that islets can be nanoencapsulated through a layer-by-layer approach using alternating layers of either poly-allylamine hydrochloride or poly-diallyldimethyl-ammonium chloride as polycations and poly-styrene sulfonate as a polyanion [177]. Subsequent studies improved the layer-by-layer coating by using a biotin-PEG peptide conjugated with streptavidin in the PEG coating [178, 179]. Nanoencapsulation of islets using alternate layers of phosphorylcholine-derived polysaccharides and alginate normalized blood sugar levels and reversed hyperglycemia in diabetic mice in an allotransplantation setting [180]. Another study demonstrated that PEGylated nanocoated islets can induce normoglycemia for more than 100 days in an allotransplantation murine model of type 1 diabetes [181].
In a recent study, it was shown that nanothin coating of islets with poly(N-vinylpyrrolidone) and tannic acid decreased pro-inflammatory chemokine synthesis and T cell migration, and restored euglycemia after allotransplantation into diabetic mice [182]. Layer-by-layer coating with three layers of PEG molecules on non-human primate islets provided a uniform nano-shielding and 100% survival rate for 150 days post-transplantation in a xenotransplantation setting in the presence of immunosuppression [183]. Despite the promising results seen with layer-by-layer coated islets in providing immunoprotection and improving graft survival in small animal models they are yet to be tested in large animal models which warrants further investigation before proceeding to clinical trials.
5. New encapsulation technologies
Several encapsulation technologies are currently being explored to improve hydrogel biocompatibility, robustness, and improve graft survival. Using electrospinning technology, highly porous hydrogel based nanofiber-enabled encapsulation devices (NEEDs) were made without altering the intrinsic chemistry of hydrogels [184]. Encapsulating rat islets within these devices did not alter islet function and were shown to reverse diabetes when transplanted into diabetic mice with minimal PFO. A California group developed a novel micro- and a nanoporous thin-film cell encapsulation device using a FDA approved polymer polycaprolactone (PCL) which allows long term bioluminescent transfer imaging (185). They demonstrated that encapsulating islets within these PCL devices did not affect islet function and rendered selective protection from immune cells and cytokines. Further, they also showed that allotransplantation of insulin producing cells encased within these thin-film devices did not elicit a foreign body response and induced neovascularization around the device. To overcome islet loss, Sabek et al. developed a novel encapsulation silicon device, the NanoGland, to prolong islet survival and promote revascularization [186]. The NanoGland device preserved islet viability and function in vitro for over 30 days and for over 120 days when transplanted subcutaneously in mice with neovascularization seen in the retrieved grafts.
A recent study tested the suitability of hyaluronic acid/collagen hydrogel as an alternative to alginate which are prone to PFO resulting in premature graft failure [187]. Islet encapsulation in hyaluronic acid/collagen hydrogels retained islet morphology and function and provided long-term immunoprotection for over 18 months in an allotransplantation setting with little or no evidence of PFO on retrieved grafts. Microfluidics based encapsulation technologies are actively being pursued by varied groups as they offer many advantages compared to conventional electro-static spraying or air-syringe pump-based encapsulation methods by producing uniform capsule size with less coefficient of variation and improved sphericity of capsules [188].
6. Tracking microcapsules
There are multiple reasons for failure of encapsulated islet grafts. These include PFO, hypoxia and erroneous delivery of microcapsules, resulting in capsule aggregation and subsequently islet starvation and islet death [189-191]. Strategies could be developed to improve clinical outcomes if microencapsulated islets infused into the peritoneal cavity could be tracked by non-invasive means to better understand the optimal delivery method, capsule distribution, and engraftment. Magnetic resonance imaging (MRI) is the most commonly used non-invasive technique for tracking cells due to its high resolution and enhanced tissue contrast [192]. A range of iron oxide nanoparticles have been employed as MRI contrast agents and especially super-paramagnetic iron oxide (SPIO) particles have been extensively studied due to their high relaxitivity and enhanced negative contrast [193]. Labeling islets with SPIO does not seem to impact viability, and SPIO nanoparticles have been used to produce magneto-capsules for encapsulation of pancreatic islets, and for non-invasive tracking by MRI [194]. However, there are major drawbacks with SPIO particles in terms of stability and magnetic sensitivity [195]. which can be overcome by using large micrometer-sized iron particles such as magnetic microspheres. Recently, we showed that islets can be labeled efficiently with these magnetic microspheres, and that encapsulated labeled islets can be tracked non-invasively by MRI [196].
Another group of contrast agents, namely perfluorocarbons (PFC), have been employed to produce fluorocapsules for microencapsulated cell tracking using 19F MRI. Furthermore, these fluorocapsules allow multimodal imaging, and can be scanned on clinical grade ultrasound and CT scanners [197]. Similarly, microcapsules loaded with gold-gadolinium nanoparticles helped to track transplanted encapsulated islets with multiple imaging modalities such as MRI, CT, and US [198]. Encapsulating contrast agents such as barium sulphate or bismuth sulphide produced radio-opaque microcapsules which can be tracked non-invasively by x-ray imaging [199]. In another study, a simple method was developed using gold nanoparticles as a contrast agent to track single alginate microcapsules using x-ray micro-CT system with a reduced radiation dose [200]. The same research group demonstrated that coating cationic homopolymer modified gold nanoparticles onto the surface of negatively charged alginate microcapsules resulted in hybrid capsules, which can be easily detected with a low X-ray dose both in vitro and in vivo [201]. However, in both studies using gold nanoparticles, the authors demonstrated the feasibility of the system only in a post-mortem rodent model, and hence warrant further investigation in live animal models.
Although the above imaging studies require further validation for clinical trials, they have clearly demonstrated that many contrast agents can be readily incorporated into the microcapsule matrix with negligible toxicity, thereby paving the way for a new generation of imaging biomaterials that can be tracked with multiple imaging modalities.
7. Encapsulation of stem cells
One of the major factors hindering islet transplantation is the limited availability of donor pancreases, i.e., access to unlimited source of insulin-producing cells. The donor shortage issue has been addressed extensively, and porcine islets have been suggested as one potential source of clinical islets [202]. Despite the risks associated with xenotransplantation seem to be reduced, recent advances in stem cell differentiation have opened up the prospect of obtaining sufficient amounts of insulin-producing cells from human sources. Studies have clearly demonstrated that fully functional beta-cells or pancreatic progenitors can be obtained from either human embryonic stem cells (hESC) [203, 204] or induced pluripotent stem cells (iPSC) [205]. Since the insulin-producing cells are obtained from a human source, and more recently from the patient's own cells, it is anticipated that there will be only a minimal alloreactivity to the transplanted cells. However, considering the underlying autoimmune etiology of type 1 diabetes, it is likely that stem cell-derived beta-cells will still need to be encased within capsules to protect from immune destruction.
Studies have shown that hESC can be encapsulated within alginate microcapsules and differentiated into definitive endoderm, and pancreatic progenitors with capsule stiffness playing a major role in regulating the efficiency of pancreatic differentiation [206-208]. ViaCyte LLC (San Diego, CA), a pioneer in hESC differentiation to pancreatic lineages, demonstrated the feasibility of encapsulating pancreatic progenitors into a macrodevice (TheraCyte) that mature into glucose-responsive insulin-producing cells and other endocrine cells upon transplantation into mice [209, 210]. Others obtained similar results showing that efficient differentiation of pancreatic progenitors can occur in a macroencapsulation device, producing mature glucose-responsive cells capable of reversing chemically induced diabetes in vivo [211, 212]. Recently, it has been shown that encapsulated neonatal pancreatic cells can be immune protected, and can reverse diabetes in a murine model of virus-induced type 1 diabetes [213]. It has also been shown that cryopreservation of macroencapsulated insulin-producing cells were safe, and that encapsulated cells were functional post-thawing [214]. ViaCyte is currently evaluating its encapsulated stem cell-derived product "VC-01" in a Phase 1/2 clinical trial for safety and efficacy, which is one of the first embryonic stem cell-derived therapies to be tested in the clinic. Initial results suggest the approach to be safe, but of limited efficacy (unpublished data).
Stem cells derived from other sources such as amniotic fluid and adipose tissue can be transformed into insulin-producing cells, encapsulated, and normalized blood sugar levels when transplanted into diabetic animal recipients [215, 216]. The incorporation of mesenchymal stem cells (MSC) and extracellular matrix (ECM) proteins into the encapsulation device matrix improved graft survival and function as the capsule microenvironment mimicked the natural pancreatic milieu [217]. In this regard, the use of sulphated alginate to encapsulate insulin producing cells might be of interest as it mimics heparan sulphate a natural component of the ECM to provide the desired microenvironment and as well for the reduction of PFO [218, 219]. These studies demonstrate that a combination of stem cells and encapsulation technologies could hold the answer to a widely applicable and effective therapy for type 1 diabetes.
8. Conclusions
In conclusion, various preclinical studies support the efficacy of alginate encapsulated islet transplantation in diabetic models and data from clinical trials suggest that they are safe, However, graft survival and function is severely hampered by the presence of PFO, leading to blockage of nutrient and oxygen transfer, resulting in islet cell hypoxia and death. The mechanism of PFO is still vague, and it is not clear whether the host immune response is directed against the encapsulating material/tissue or both. However, recent findings in reducing PFO by alginate purification, altering microcapsule geometry, modifying alginate chemical composition, co-encapsulation with immunomodulatory agents/cells, and new encapsulation technologies provide an optimistic view of the future of encapsulated islet transplantation.
With the recent developments in porcine islet transplantation and deriving human insulin-producing cells from varied stem cells, the outlook for encapsulated insulin-producing tissue looks encouraging both short- and long-term. Ongoing clinical trials will provide further insight into the safety and efficacy of encapsulated insulin producing cell product, although a concerted research effort is required for its clinical translation. Finally, guidelines and consensus for the transplantation of encapsulated insulin-producing cells sourced from pancreas (human/porcine) or stem cells (hESC/iPSC) need to be framed, which might have consequences on the future of encapsulated islet transplantation.
Disclosures: The authors report no conflict of interests.
Appendix
Table
1.
Summary of varied strategies adopted to prevent/reduce PFO and improve encapsulated islet survival |
|
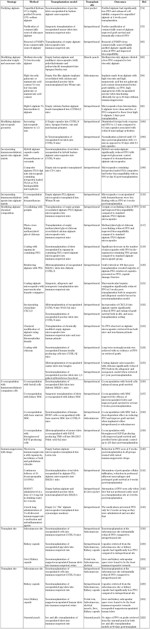 |
 |
Acknowledgments:
We wish to thank Prof. Liangxue Lai and his team at Guangzhou Institute of Biomedicine and Health for transplanting and recovering alginate microcapsules in pigs.
References
- Tisch R, McDevitt H. Insulin-dependent diabetes mellitus. Cell 1996. 85(3):291-297. [DOD] [CrossRef]
- Cavan D, Fernandes JR, Makaroff L, Ogurtsova K, Webber S. IDF Diabetes Atlas. International Diabetes Federation 2015. Chapter 3, pp 47-63. [DOD]
- van Belle TL, Coppieters KT, von Herrath MG. Type 1 diabetes: etiology, immunology, and therapeutic strategies. Physiol Rev 2011. 91(1):79-118. [DOD] [CrossRef]
- Tanenberg RJ, Newton CA, Drake AJ. Confirmation of hypoglycemia in the "dead-in-bed" syndrome, as captured by a retrospective continuous glucose monitoring system. Endocr Pract 2010. 16(2):244-248. [DOD] [CrossRef]
- Gruessner AC, Sutherland DE. Pancreas transplant outcomes for United States (US) cases as reported to the United Network for Organ Sharing (UNOS) and the International Pancreas Transplant Registry (IPTR). Clin Transpl 2008. 2008:45-56. [DOD]
- Jahansouz C, Kumer SC, Ellenbogen M, Brayman KL. Evolution of beta-Cell Replacement Therapy in Diabetes Mellitus: Pancreas Transplantation. Diabetes Technol Ther 2011. 13(3):395-418. [DOD] [CrossRef]
- Gruessner AC. Update on pancreas transplantation: comprehensive trend analysis of 25,000 cases followed up over the course of twenty-four years at the International Pancreas Transplant Registry (IPTR). Rev Diabet Stud 2011. 8(1):6-16. [DOD] [CrossRef]
- Johnson PR, Jones KE. Pancreatic islet transplantation. Semin Pediatr Surg 2012. 21(3):272-280. [DOD] [CrossRef]
- Shapiro AMJ, Lakey JR, Ryan EA, Korbutt GS, Toth E, Warnock GL, Kneteman NM, Rajotte RV. Islet transplantation in seven patients with type 1 diabetes mellitus using a glucocorticoid-free immunosuppressive regimen. N Engl J Med 2000. 343(4):230-238. [DOD] [CrossRef]
- Barton FB, Rickels MR, Alejandro R, Hering BJ, Wease S, Naziruddin B, Oberholzer J, Odorico JS, Garfinkel MR, Levy M, et al. Improvement in outcomes of clinical islet transplantation: 1999-2010. Diabetes Care 2012. 35(7):1436-1445. [DOD] [CrossRef]
- Appel M, Hering B. CITR Seventh Annual Report. Collaborative Islet Transplant Registry 2010. Chapter 1, pp 1-13. [DOD]
- Gibly RF, Graham JG, Luo X, Lowe WL Jr, Hering BJ, Shea LD. Advancing islet transplantation: from engraftment to the immune response. Diabetologia 2011. 54(10):2494-2505. [DOD] [CrossRef]
- Carlsson PO, Palm F, Andersson A, Liss P. Markedly decreased oxygen tension in transplanted rat pancreatic islets irrespective of the implantation site. Diabetes 2001. 50(3):489-495. [DOD] [CrossRef]
- Bennet W, Sundberg B, Groth CG, Brendel MD, Brandhorst D, Brandhorst H, Bretzel RG, Elgue G, Larsson R, Nilsson B, et al. Incompatibility between human blood and isolated islets of Langerhans: a finding with implications for clinical intraportal islet transplantation? Diabetes 1999. 48(10):1907-1914. [DOD]
- Bell E, Cao X, Moibi JA, Greene SR, Young R, Trucco M, Gao Z, Matschinsky FM, Deng S, Markman JF, et al. Rapamycin has a deleterious effect on MIN-6 cells and rat and human islets. Diabetes 2003. 52(11):2731-2739. [DOD] [CrossRef]
- Vincenti F, Friman S, Scheuermann E, Rostaing L, Jenssen T, Campistol JM, Uchida K, Pescovitz MD, Marchetti P, Tuncer M, et al. DIRECT (Diabetes Incidence after Renal Transplantation: Neoral C Monitoring Versus Tacrolimus) Investigators. Results of an international, randomized trial comparing glucose metabolism disorders and outcome with cyclosporine versus tacrolimus. Am J Transplant 2007. 7(6):1506-1514. [DOD] [CrossRef]
- Ichii H, Ricordi C. Current status of islet cell transplantation. J Hepatobiliary Pancreat Surg 2009. 16(2):101-112. [DOD] [CrossRef]
- Lanza RP, Hayes JL, Chick WL. Encapsulated cell technology. Nat Biotechnol 1996. 14(9):1107-1111. [DOD] [CrossRef]
- Sun AM, Parisius W, Healy GM, Vacek I, Macmorine HG. The use, in diabetic rats and monkeys, of artificial capillary units containing cultured islets of Langerhans (artificial endocrine pancreas). Diabetes 1977. 26(12):1136-1139. [DOD] [CrossRef]
- Maki T, Lodge JP, Carretta M, Ohzato H, Borland KM, Sullivan SJ, Staruk J, Muller TE, Solomon BA, Chick WL. Treatment of severe diabetes mellitus for more than one year using a vascularized hybrid artificial pancreas. Transplantation 1993. 55(4):713-717. [DOD] [CrossRef]
- Hwang PTJ, Shah DK, Garcia JA, Bae CY, Lim D, Huiszoon RC, Alexander GC, Jun H. Progress and challenges of the bioartificial pancreas. Nano Converg 2016. 3(1):28. [DOD] [CrossRef]
- Song S, Faleo G, Yeung R, Kant R, Posselt AM, Desai TA, Tang Q, Roy S. Silicon nanopore membrane (SNM) for islet encapsulation and immunoisolation under convective transport. Sci Rep 2016. 24;6:23679. [DOD] [CrossRef]
- Scharp DW, Swanson CJ, Olack BJ, Latta PP, Hegre OD, Doherty EJ, Gentile FT, Flavin KS, Ansara MF, Lacy PE. Protection of encapsulated human islets implanted without immunosuppression in patients with type I or type II diabetes and in nondiabetic control subjects. Diabetes 1994. 43(9):1167-1170. [DOD] [CrossRef]
- Colton CK. Implantable biohybrid artificial organs. Cell Transplant 1995. 4(4):415-436. [DOD] [CrossRef]
- Storrs R, Dorian R, King SR, Lakey J, Rilo H. Preclinical development of the Islet Sheet. Ann N Y Acad Sci 2001. 944:252-266. [DOD] [CrossRef]
- Lamb M, Storrs R, Li S, Liang O, Laugenour K, Dorian R, Chapman D, Ichii H, Imagawa D, Foster C, et al. Function and viability of human islets encapsulated in alginate sheets: in vitro and in vivo culture. Transplant Proc 2011. 43(9):3265-3266. [DOD] [CrossRef]
- Boettler T, Schneider D, Cheng Y, Kadoya K, Brandon EP, Martinson L, von Herrath M. Pancreatic tissue transplanted in theracyte encapsulation devices is protected and prevents hyperglycemia in a mouse model of immune-mediated diabetes. Cell Transplant 2016. 25(3):609-614. [DOD] [CrossRef]
- Sorenby AK, Kumagai-Braesch M, Sharma A, Hultenby KR, Wernerson AM, Tibell AB. Preimplantation of an immunoprotective device can lower the curative dose of islets to that of free islet transplantation: studies in a rodent model. Transplantation 2008. 86(2):364-366. [DOD] [CrossRef]
- Kumagai-Braesch M, Jacobson S, Mori H, Jia X, Takahashi T, Wernerson A, Flodström-Tullberg M, Tibell A. The TheraCyte™ device protects against islet allograft rejection in immunized hosts. Cell Transplant 2013. 22(7):1137-1146. [DOD] [CrossRef]
- Bartlett ST, Markmann JF, Johnson P, Korsgren O, Hering BJ, Scharp D, Kay TW, Bromberg J, Odorico JS, Weir GC, et al. Report from IPITA-TTS Opinion Leaders Meeting on the Future of β-Cell Replacement. Transplantation 2016. 100(2):S1-S44. [DOD] [CrossRef]
- Ludwig B, Rotem A, Schmid J, Weir GC, Colton CK, Brendel MD, Neufeld T, Block NL, Yavriyants K, Steffen A, et al. Improvement of islet function in a bioartificial pancreas by enhanced oxygen supply and growth hormone releasing hormone agonist. Proc Natl Acad Sci U S A 2012. 27;109(13):5022-5027. [DOD] [CrossRef]
- Neufeld T, Ludwig B, Barkai U, Weir GC, Colton CK, Evron Y, Balyura M, Yavriyants K, Zimermann B, Azarov D, et al. The efficacy of an immunoisolating membrane system for islet xenotransplantation in minipigs. PLoS One 2013. 8(8):e70150. [DOD] [CrossRef]
- Ludwig B, Reichel A, Steffen A, Zimerman B, Schally AV, Block NL, Colton CK, Ludwig S, Kersting S, Bonifacio E, et al. Transplantation of human islets without immunosuppression. Proc Natl Acad Sci U S A 2013. 110(47):19054-19058. [DOD] [CrossRef]
- van Schilfgaarde R, de Vos P. Factors influencing the properties and performance of microcapsules for immunoprotection of pancreatic islets. J Mol Med 1999. 77(1):199-205. [DOD] [CrossRef]
- Spector M, Cease C, Tong-Li X. The local tissue response to biomaterials. Crit Rev Biocomp 1989. 5:269-295. [DOD]
- de Vos P, Lazarjani HA, Poncelet D, Faas MM. Polymers in cell encapsulation from an enveloped cell perspective. Adv Drug Deliv Rev 2014. 67-68:15-34. [DOD] [CrossRef]
- de Vos P, Faas MM, Strand B, Calafiore R. Alginate-based microcapsules for immunoisolation of pancreatic islets. Biomaterials 2006. 27(32):5603-5617. [DOD] [CrossRef]
- Strand BL, Morch YA, Skjak-Braek G. Alginate as immobilization matrix for cells. Minerva Biotechnologica 2000. 12(4):223-233. [DOD]
- Lim F, Sun AM. Microencapsulated islets as bioartificial endocrine pancreas. Science 1980. 210(4472):908-910. [DOD] [CrossRef]
- de Vos P, De Haan B, Wolters GH, van Schilfgaarde R. Factors influencing the adequacy of microencapsulation of rat pancreatic islets. Transplantation 1996. 62(7):888-893. [DOD] [CrossRef]
- Robitaille R, Dusseault J, Henley N, Desbiens K, Labrecque N, Hallé JP. Inflammatory response to peritoneal implantation of alginate-poly-L-lysine microcapsules. Biomaterials 2005. 26(19):4119-4127. [DOD] [CrossRef]
- Kulseng B, Skjåk-Braek G, Ryan L, Andersson A, King A, Faxvaag A, Espevik T. Transplantation of alginate microcapsules: generation of antibodies against alginates and encapsulated porcine islet-like cell clusters. Transplantation 1999. 67(7):978-984. [DOD] [CrossRef]
- Silva AI, de Matos AN, Brons IG, Mateus M. An overview on the development of a bio-artificial pancreas as a treatment of insulin-dependent diabetes mellitus. Med Res Rev 2006. 26(2):181-222. [DOD] [CrossRef]
- Elliott RB, Escobar L, Tan PL, Garkavenko O, Calafiore R, Basta P, Vasconcellos AV, Emerich DF, Thanos C, Bambra C. Intraperitoneal alginate-encapsulated neonatal porcine islets in a placebo-controlled study with 16 diabetic cynomolgus primates. Transplant Proc 2005. 37(8):3505-3508. [DOD] [CrossRef]
- Duvivier-Kali VF, Omer A, Parent RJ, O'Neil JJ, Weir GC. Complete protection of islets against allorejection and autoimmunity by a simple barium-alginate membrane. Diabetes 2001. 50(8):1698-1705. [DOD] [CrossRef]
- van Raamsdonk JM, Cornelius RM, Brash JL, Chang PL. Deterioration of polyamino acid-coated alginate microcapsules in vivo. J Biomater Sci Polym Ed 2002. 13(8):863-884. [DOD] [CrossRef]
- Morch YA, Donati I, Strand BL, Skjak-Braek G. Effect of Ca2+, Ba2+, and Sr2+ on alginate microbeads. Biomacromolecules 2006. 7(5):1471-1480. [DOD] [CrossRef]
- Omer A, Duvivier-Kali VF, Trivedi N, Wilmot K, Bonner-Weir S, Weir GC. Survival and maturation of microencapsulated porcine neonatal pancreatic cell clusters transplanted into immunocompetent diabetic mice. Diabetes 2003. 52(1):69-75. [DOD] [CrossRef]
- Foster JL, Williams G, Williams LJ, Tuch BE. Differentiation of transplanted microencapsulated fetal pancreatic cells. Transplantation 2007. 83(11):1440-1448. [DOD] [CrossRef]
- Vaithilingam V, Oberholzer J, Guillemin GJ, Tuch BE. The humanized NOD/SCID mouse as a preclinical model to study the fate of encapsulated human islets. Rev Diabet Stud 2010. 7(1):62-73. [DOD] [CrossRef]
- Vaithilingam V, Fung C, Ratnapala S, Foster J, Vaghjiani V, Manuelpillai U, Tuch BE. Characterisation of the xenogeneic immune response to microencapsulated fetal pig islet-like cell clusters transplanted into immunocompetent C57BL/6 mice. PLoS One 2013. 8(3):e59120. [DOD] [CrossRef]
- Robles L, Storrs R, Lamb M, Alexander M, Lakey JR. Current status of islet encapsulation. Cell Transplant 2014. 23(11):1321-1348. [DOD] [CrossRef]
- Vaithilingam V, Kollarikova G, Qi M, Lacik I, Oberholzer J, Guillemin GJ, Tuch BE. Effect of prolonged gelling time on the intrinsic properties of barium alginate microcapsules and its biocompatibility. J Microencapsul 2011. 28(6):499-507. [DOD] [CrossRef]
- de Vos P, van Hoogmoed CG, van Zanten J, Netter S, Strubbe JH, Busscher HJ. Long-term biocompatibility, chemistry, and function of microencapsulated pancreatic islets. Biomaterials 2003. 24(2):305-312. [DOD] [CrossRef]
- Qi M, Lacik I, Kolláriková G, Strand BL, Formo K, Wang Y, Marchese E, Mendoza-Elias JE, Kinzer KP, Gatti F, et al. A recommended laparoscopic procedure for implantation of microcapsules in the peritoneal cavity of non-human primates. J Surg Res 2011. 168(1):e117-e123. [DOD] [CrossRef]
- Toso C, Oberholzer J, Ceausoglu I, Ris F, Rochat B, Rehor A, Bucher P, Wandrey C, Schuldt U, Belenger J, et al. Intra-portal injection of 400-microm microcapsules in a large-animal model. Transpl Int 2003. 16(6):405-410. [DOD]
- Soon-Shiong P, Heintz RE, Merideth N, Yao QX, Yao Z, Zheng T, Murphy M, Moloney MK, Schmehl M, Harris M, et al. Insulin independence in a type 1 diabetic patient after encapsulated islet transplantation. Lancet 1994. 343(8903):950-951. [DOD] [CrossRef]
- Calafiore R, Basta G, Luca G, Lemmi A, Montanucci MP, Calabrese G, Racanicchi L, Mancuso F, Brunetti P. Microencapsulated pancreatic islet allografts into nonimmunosuppressed patients with type 1 diabetes: first two cases. Diabetes Care 2006. 29(1):137-138. [DOD] [CrossRef]
- Basta G, Montanucci P, Luca G, Boselli C, Noya G, Barbaro B, Qi M, Kinzer KP, Oberholzer J, Calafiore R. Long-term metabolic and immunological follow-up of non-immunosuppressed patients with type 1 diabetes treated with microencapsulated islet allografts: four cases. Diabetes Care 2011. 34(11):2406-2409. [DOD] [CrossRef]
- Elliott RB, Escobar L, Tan PL, Muzina M, Zwain S, Buchanan C. Live encapsulated porcine islets from a type 1 diabetic patient 9.5 yr after xenotransplantation. Xenotransplantation 2007. 14(2):157-161. [DOD] [CrossRef]
- Elliott RB. Microencapsulated neonatal porcine islet implants alleviate unaware hypoglycaemia without immune suppression. 13th World Congress of the IPITA, Prague, Jun 1-4, 2011. [DOD]
- Matsumoto S, Abalovich A, Wechsler C, Wynyard S, Elliott RB. Clinical benefit of islet xenotransplantation for the treatment of type 1 diabetes. EBioMedicine 2016. 12:255-262. [DOD] [CrossRef]
- Tuch BE, Keogh GW, Williams LJ, Wu W, Foster JL, Vaithilingam V, Philips R. Safety and viability of microencapsulated human islets transplanted into diabetic humans. Diabetes Care 2009. 32(10):1887-1889. [DOD] [CrossRef]
- Jacobs-Tulleneers-Thevissen D, Chintinne M, Ling Z, Gillard P, Schoonjans L, Delvaux G, Strand BL, Gorus F, Keymeulen B, Pipeleers D, Beta Cell Therapy Consortium EU-FP7. Sustained function of alginate-encapsulated human islet cell implants in the peritoneal cavity of mice leading to a pilot study in a type 1 diabetic patient. Diabetologia 2013. 56(7):1605-1614. [DOD]
- Espevik T, Rokstad AM, Kulseng B, Strand B, Skjak-Braek G. Mechansim of host immune response to alginate microcapsules. In: Halle JP, de Vos P, Rosenberg L. The Bioartificial Pancreas and Other Biohybrid Therapies. Transworld Research 2009. pp 279-290. [DOD]
- Ludwig B, Ludwig S. Transplantable bioartificial pancreas devices: current status and future prospects. Langenbecks Arch Surg 2015. 400(5):531-540. [DOD] [CrossRef]
- Kepsutlu B, Nazli C, Bal T, Kizilel S. Design of bioartificial pancreas with functional micro/nano-based encapsulation of islets. Curr Pharm Biotechnol 2014. 15(7):590-608. [DOD] [CrossRef]
- Cooper DK, Matsumoto S, Abalovich A, Itoh T, Mourad NI, Gianello PR, Wolf E, Cozzi E. Progress in clinical encapsulated islet xenotransplantation. Transplantation 2016. 100(11):2301-2308. [DOD] [CrossRef]
- Bakeine GJ, Bertolotti A, Latina M, Congiu T, Prati U, Roveda L, Trotta F, Tormen M, Fabrizio ED, Carlini G, et al. Surface properties and implantation site affect the capsular fibrotic overgrowth. J Biomed Mater Res A 2007. 83(4):965-969. [DOD] [CrossRef]
- O'Sullivan ES, Vegas A, Anderson DG, Weir GC. Islets transplanted in immunoisolation devices: a review of the progress and the challenges that remain. Endocr Rev 2011. 32(6):827-844. [DOD] [CrossRef]
- Horcher A, Zekorn T, Siebers U, Klöck G, Frank H, Houben R, Bretzel RG, Zimmermann U, Federlin K. Transplantation of microencapsulated islets in rats: evidence for induction of fibrotic overgrowth by islet alloantigens released from microcapsules. Transplant Proc 1994. 26(2):784-786. [DOD]
- Skjak-Braek G, Murano E, Paoletti S. Alginate as immobilization material. II: Determination of polyphenol contaminants by fluorescence spectroscopy, and evaluation of methods for their removal. Biotechnol Bioeng 1989. 33(1):90-94. [DOD] [CrossRef]
- Kawai T, Akira S. The role of pattern recognition receptors in innate immunity: update on Toll-like receptors. Nat Immunol 2010. 11(5):373-384. [DOD] [CrossRef]
- Zhang WJ, Laue C, Hyder A, Schrezenmeir J. Purity of alginate affects the viability and fibrotic overgrowth of encapsulated porcine islet xenografts. Transplant Proc 2001. 33(7-8):3517-3519. [DOD] [CrossRef]
- Dusseault J, Tam SK, Ménard M, Polizu S, Jourdan G, Yahia L, Hallé JP. Evaluation of alginate purification methods: effect on polyphenol, endotoxin, and protein contamination. J Biomed Mater Res A 2006. 76(2):243-251. [DOD] [CrossRef]
- Mallett AG, Korbutt GS. Alginate modification improves long-term survival and function of transplanted encapsulated islets. Tissue Eng Part A 2009. 15(6):1301-1309. [DOD] [CrossRef]
- de Haan BJ, Rossi A, Faas MM, Smelt MJ, Sonvico F, Colombo P, de Vos P. Structural surface changes and inflammatory responses against alginate-based microcapsules after exposure to human peritoneal fluid. J Biomed Mater Res A 2011. 98(3):394-403. [DOD] [CrossRef]
- de Vos P, Smedema I, van Goor H, Moes H, van Zanten J, Netters S, de Leij LF, de Haan A, de Haan BJ. Association between macrophage activation and function of micro-encapsulated rat islets. Diabetologia 2003. 46(5):666-673. [DOD] [CrossRef]
- Halle JP, Bourassa S, Leblond FA, Chevalier S, Beaudry M, Chapdelaine A, Cousineau S, Saintonge J, Yale JF. Protection of islets of Langerhans from antibodies by microencapsulation with alginate-poly-L-lysine membranes. Transplantation 1993. 55(2):350-354. [DOD] [CrossRef]
- Paredes-Juarez GA, de Haan BJ, Faas MM, de Vos P. A technology platform to test the efficacy of purification of alginate. Materials 2014. 7(3):2087-2103. [DOD] [CrossRef]
- Juste S, Lessard M, Henley N, Ménard M, Hallé JP. Effect of poly-L-lysine coating on macrophage activation by alginate-based microcapsules: assessment using a new in vitro method. J Biomed Mater Res A 2005. 72(4):389-398. [DOD] [CrossRef]
- Nafea EH, Marson A, Poole-Warren LA, Martens PJ. Immunoisolating semi-permeable membranes for cell encapsulation: focus on hydrogels. J Control Release 2011. 154(2):110-122. [DOD] [CrossRef]
- Paredes-Juarez GA, de Haan BJ, Faas MM, de Vos P. The role of pathogen-associated molecular patterns in inflammatory responses against alginate based microcapsules. J Control Release 2013. 172(3):983-992. [DOD] [CrossRef]
- de Vos P, Bucko M, Gemeiner P, Navrátil M, Svitel J, Faas M, Strand BL, Skjak-Braek G, Morch YA, Vikartovská A, et al. Multiscale requirements for bioencapsulation in medicine and biotechnology. Biomaterials 2009. 30(13):2559-2570. [DOD] [CrossRef]
- Calafiore R, Basta G. Clinical application of microencapsulated islets: actual prospectives on progress and challenges. Adv Drug Deliv Rev 2014. 67-68:84-92. [DOD] [CrossRef]
- de Groot M, Schuurs TA, van Schilfgaarde R. Causes of limited survival of microencapsulated pancreatic islet grafts. J Surg Res 2004. 121(1):141-150. [DOD] [CrossRef]
- Ertesvag H, Valla S. Biosynthesis and applications of alginates. Polym Degrad Stability 1998. 59(1-3):85-91. [DOD] [CrossRef]
- Kulseng B, Skjak-Braek G, Ryan L, Andersson A, King A, Faxvaag A, Espevik T. Transplantation of alginate microcapsules: generation of antibodies against alginates and encapsulated porcine islet-like cell clusters. Transplantation 1999. 67(7):978-984. [DOD] [CrossRef]
- Soon-Shiong P, Otterlie M, Skjak-Braek G, Smidsrod O, Heintz R, Lanza RP, Espevik T. An immunologic basis for the fibrotic reaction to implanted microcapsules. Transplant Proc 1991. 23(1):758-759. [DOD]
- Clayton HA, London NJ, Colloby PS, Bell PR, James RF. The effect of capsule composition on the biocompatibility of alginate-poly-l-lysine capsules. J Microencapsul 1991. 8(2):221-233. [DOD] [CrossRef]
- de Vos P, de Haan B, van Schilfgaarde R. Effect of the alginate composition on the biocompatibility of alginate-polylysine microcapsules. Biomaterials 1997. 18(3):273-278. [DOD] [CrossRef]
- Tam SK, Dusseault J, Bilodeau S, Langlois G, Hallé JP, Yahia L. Factors influencing alginate gel biocompatibility. J Biomed Mater Res A 2011. 98(1):40-52. [DOD] [CrossRef]
- Schneider S, Feilen PJ, Kraus O, Haase T, Sagban TA, Lehr HA, Beyer J, Pommersheim R, Weber MM. Biocompatibility of alginates for grafting: impact of alginate molecular weight. Artif Cells Blood Substit Immobil Biotechnol 2003. 31(4):383-394. [DOD] [CrossRef]
- Veriter S, Mergen J, Goebbels RM, Aouassar N, Gregoire C, Jordan B, Levêque P, Gallez B, Gianello P, Dufrane D. In vivo selection of biocompatible alginates for islet encapsulation and subcutaneous transplantation. Tissue Eng Part A 2010. 16(5):1503-1513. [DOD] [CrossRef]
- Williams SJ, Huang HH, Kover K, Moore W, Berkland C, Singh M, Smirnova IV, MacGregor R, Stehno-Bittel L. Reduction of diffusion barriers in isolated rat islets improves survival, but not insulin secretion or transplantation outcome. Organogenesis 2010. 6(2):115-124. [DOD] [CrossRef]
- Chicheportiche D, Reach G. In vitro kinetics of insulin release by microencapsulated rat islets: effect of the size of the microcapsules. Diabetologia 1988. 31(1):54-57. [DOD]
- Schrezenmeir J, Gerö L, Laue C, Kirchgessner J, Müller A, Hüls A, Passmann R, Hahn HJ, Kunz L, Mueller-Klieser W, et al. The role of oxygen supply in islet transplantation. Transplant Proc 1992. 24(6):2925-2929. [DOD]
- Robitaille R, Pariseau JF, Leblond FA, Lamoureux M, Lepage Y, Hallé JP. Studies on small (< 350 microm) alginate-poly-L-lysine microcapsules. III. Biocompatibility Of smaller versus standard microcapsules. J Biomed Mater Res 1999. 44(1):116-120. [DOD] [CrossRef]
- Lum ZP, Krestow M, Tai IT, Vacek I, Sun AM. Xenografts of rat islets into diabetic mice. An evaluation of new smaller capsules. Transplantation 1992. 53(6):1180-1183. [DOD] [CrossRef]
- Veiseh O, Doloff JC, Ma M, Vegas AJ, Tam HH, Bader AR, Li J, Langan E, Wyckoff J, Loo WS, et al. Size- and shape-dependent foreign body immune response to materials implanted in rodents and non-human primates. Nat Mater 2015. 14(6):643-651. [DOD] [CrossRef]
- Thu B, Bruheim P, Espevik T, Smidsrod O, Soon-Shiong P, Skjak-Braek G. Alginate polycation microcapsules. I. Interaction between alginate and polycation. Biomaterials 1996. 17(10):1031-1040. [DOD] [CrossRef]
- Strand BL, Ryan TL, Veld P, Kulseng B, Rokstad AM, Skjak-Brek G, Espevik T. Poly-L-Lysine induces fibrosis on alginate microcapsules via the induction of cytokines. Cell Transplant 2001. 10(3):263-275. [DOD] [CrossRef]
- King A, Sandler S, Andersson A. The effect of host factors and capsule composition on the cellular overgrowth on implanted alginate capsules. J Biomed Mater Res 2001. 57(3):374-383. [DOD] [CrossRef]
- Tam SK, Bilodeau S, Dusseault J, Langlois G, Hallé JP, Yahia LH. Biocompatibility and physicochemical characteristics of alginate-polycation microcapsules. Acta Biomater 2011. 7(4):1683-1692. [DOD] [CrossRef]
- Orive G, Bartkowiak A, Lisiecki S, De Castro M, Hernandez RM, Gascon AR, Pedraz JL. Biocompatible oligochitosans as cationic modifiers of alginate/Ca microcapsules. J Biomed Mater Res B Appl Biomater 2005. 74(1):429-439. [DOD] [CrossRef]
- Bystricky S, Malovíkova A, Sticzay T. Interaction of acidic polysaccharides with polylysine enantiomers. Conformation probe in solution. Carbohydr Polym 1991. 15(3):299-308. [DOD] [CrossRef]
- Spasojevic M, Bhujbal S, Paredes G, de Haan BJ, Schouten AJ, de Vos P. Considerations in binding diblock copolymers on hydrophilic alginate beads for providing an immunoprotective membrane. J Biomed Mater Res A 2013. 102(6):1887-1896. [DOD] [CrossRef]
- Dang TT, Thai AV, Cohen J, Slosberg JE, Siniakowicz K, Doloff JC, Ma M, Hollister-Lock J, Tang KM, Gu Z, et al. Enhanced function of immuno-isolated islets in diabetes therapy by co-encapsulation with an anti-inflammatory drug. Biomaterials 2013. 34(23):5792-5801. [DOD] [CrossRef]
- Ricci M, Blasi P, Giovagnoli S, Rossi C, Macchiarulo G, Luca G, Basta G, Calafiore R. Ketoprofen controlled release from composite microcapsules for cell encapsulation: effect on post-transplant acute inflammation. J Control Release 2005. 107(3):395-407. [DOD] [CrossRef]
- Bünger CM, Tiefenbach B, Jahnke A, Gerlach C, Freier T, Schmitz KP, Hopt UT, Schareck W, Klar E, de Vos P. Deletion of the tissue response against alginate-pll capsules by temporary release of co-encapsulated steroids. Biomaterials 2005. 26(15):2353-2360. [DOD] [CrossRef]
- Azadi SA, Vasheghani-Farahani E, Hashemi-Najafbabadi S, Godini A. Co-encapsulation of pancreatic islets and pentoxifylline in alginate-based microcapsules with enhanced immunosuppressive effects. Prog Biomater 2016. 5:101-109. [DOD] [CrossRef]
- Mooranian A, Tackechi R, Jamieson E, Morahan G, Al-Salami H. Innovative microcapsules for pancreatic β-Cells harvested from mature double-transgenic mice: Cell imaging, viability, induced glucose-stimulated insulin measurements and proinflammatory cytokines analysis. Pharm Res 2017. 34(6):1217-1223. [DOD] [CrossRef]
- Mooranian A, Negrulj R, Al-Salami H. The influence of stabilized deconjugated ursodeoxycholic acid on polymer-hydrogel system of transplantable NIT-1 cells. Pharm Res 2016. 33(5):1182-1190. [DOD] [CrossRef]
- Jo EH, Hwang YH, Lee DY. Encapsulation of pancreatic islet with HMGB1 fragment for attenuating inflammation. Biomater Res 2015. 19:21. [DOD] [CrossRef]
- Zheng J, Xie H, Yu W, Tan M, Gong F, Liu X, Wang F, Lv G, Liu W, Zheng G, et al. Enhancement of surface graft density of MPEG on alginate/chitosan hydrogel microcapsules for protein repellency. Langmuir 2012. 28(37):13261-13273. [DOD] [CrossRef]
- Hillberg AL, Kathirgamanathan K, Lam JB, Law LY, Garkavenko O, Elliott RB. Improving alginate-poly-L-ornithine-alginate capsule biocompatibility through genipin crosslinking. J Biomed Mater Res B Appl Biomater 2013. 101(2):258-268. [DOD] [CrossRef]
- Hillberg AL, Oudshoorn M, Lam JB, Kathirgamanathan K. Encapsulation of porcine pancreatic islets within an immunoprotective capsule comprising methacrylated glycol chitosan and alginate. J Biomed Mater Res B Appl Biomater 2015. 103(3):503-518. [DOD] [CrossRef]
- Orive G, Hernandez RM, Gascon AR, Igartua M, Pedraz JL. Development and optimisation of alginate-PMCG-alginate microcapsules for cell immobilisation. Int J Pharm 2003. 259(1-2):57-68. [DOD] [CrossRef]
- Park HS, Kim JW, Lee SH, Yang HK, Ham DS, Sun CL, Hong TH, Khang G, Park CG, Yoon KH. Antifibrotic effect of rapamycin containing polyethylene glycol-coated alginate microcapsule in islet xenotransplantation. J Tissue Eng Regen Med 2011. 11(4):1274-1284. [DOD] [CrossRef]
- Villa C, Manzoli V, Abreu MM, Verheyen CA, Seskin M, Najjar M, Molano RD, Torrente Y, Ricordi C, Tomei AA. Effects of composition of alginate-polyethylene glycol microcapsules and transplant site on encapsulated islet graft outcomes in mice. Transplantation 2017. 101(5):1025-1035. [DOD] [CrossRef]
- Vaithilingam V, Kollarikova G, Qi M, Larsson R, Lacik I, Formo K, Marchese E, Oberholzer J, Guillemin GJ, Tuch BE. Beneficial effects of coating alginate microcapsules with macromolecular heparin conjugates-in vitro and in vivo study. Tissue Eng Part A 2014. 20(1-2):324-334. [DOD] [CrossRef]
- Chen T, Yuan J, Duncanson S, Hibert ML, Kodish BC, Mylavaganam G, Maker M, Li H, Sremac M, Santosuosso M, et al. Alginate encapsulant incorporating CXCL12 supports long-term allo- and xenoislet transplantation without systemic immune suppression. Am J Transplant 2015. 15(3):618-627. [DOD] [CrossRef]
- Vegas AJ, Veiseh O, Doloff JC, Ma M, Tam HH, Bratlie K, Li J, Bader AR, Langan E, Olejnik K, et al. Combinatorial hydrogel library enables identification of materials that mitigate the foreign body response in primates. Nat Biotechnol 2016. 34(3):345-352. [DOD] [CrossRef]
- Vegas AJ, Veiseh O, Gürtler M, Millman JR, Pagliuca FW, Bader AR, Doloff JC, Li J, Chen M, Olejnik K, et al. Long-term glycemic control using polymer-encapsulated human stem cell-derived beta cells in immune-competent mice. Nat Med 2016. 22(3):306-311. [DOD] [CrossRef]
- Yang HK, Ham DS, Park HS, Rhee M, You YH, Kim MJ, Shin J, Kim OY, Khang G, Hong TH, et al. Long-term efficacy and biocompatibility of encapsulated islet transplantation with chitosan-coated alginate capsules in mice and canine models of diabetes. Transplantation 2016. 100(2):334-343. [DOD] [CrossRef]
- Charles R, Lu L, Qian S, Fung JJ. Stromal cell-based immunotherapy in transplantation. Immunotherapy 2011. 3(12):1471-1485. [DOD] [CrossRef]
- Korbutt GS, Elliott JF, Rajotte RV. Co-transplantation of allogeneic islets with allogeneic testicular cell aggregates allows long-term graft survival without systemic immunosuppression. Diabetes 1997. 46(2):317-322. [DOD] [CrossRef]
- Dufour JM, Rajotte RV, Kin T, Korbutt GS. Immunoprotection of rat islet xenografts by cotransplantation with sertoli cells and a single injection of antilymphocyte serum. Transplantation 2003. 75(9):1594-1596. [DOD] [CrossRef]
- Korbutt GS, Suarez-Pinzon WL, Power RF, Rajotte RV, Rabinovitch A. Testicular Sertoli cells exert both protective and destructive effects on syngeneic islet grafts in non-obese diabetic mice. Diabetologia 2000. 43(4):474-480. [DOD] [CrossRef]
- Yang H, Wright JR. Co-encapsulation of Sertoli enriched testicular cell fractions further prolongs fish-to-mouse islet xenograft survival. Transplantation 1999. 67(6):815-820. [DOD] [CrossRef]
- Luca G, Calafiore R, Basta G, Ricci M, Calvitti M, Neri L, Nastruzzi C, Becchetti E, Capitani S, Brunetti P, et al. Improved function of rat islets upon co-microencapsulation with Sertoli's cells in alginate/poly-L-ornithine. AAPS Pharm Sci Tech 2001. 2(3):E15. [DOD] [CrossRef]
- Soleymaninejadian E, Pramanik K, Samadian E. Immunomodulatory properties of mesenchymal stem cells: cytokines and factors. Am J Reprod Immunol 2012. 67(1):1-8. [DOD] [CrossRef]
- Rasmusson I. Immune modulation by mesenchymal stem cells. Exp Cell Res 2006. 312(12):2169-2179. [DOD] [CrossRef]
- Ding Y, Xu D, Feng G, Bushell A, Muschel RJ, Wood KJ. Mesenchymal stem cells prevent the rejection of fully allogenic islet grafts by the immunosuppressive activity of matrix metalloproteinase-2 and -9. Diabetes 2009. 58(8):1797-1806. [DOD] [CrossRef]
- Solari MG, Srinivasan S, Boumaza I, Unadkat J, Harb G, Garcia-Ocana A, Feili-Hariri M. Marginal mass islet transplantation with autologous mesenchymal stem cells promotes long-term islet allograft survival and sustained normoglycemia. J Autoimmun 2009. 32(2):116-124. [DOD] [CrossRef]
- Longoni B, Szilagyi E, Quaranta P, Paoli GT, Tripodi S, Urbani S, Mazzanti B, Rossi B, Fanci R, Demontis GC, et al. Mesenchymal stem cells prevent acute rejection and prolong graft function in pancreatic islet transplantation. Diabetes Technol Ther 2010. 12(6):435-446. [DOD] [CrossRef]
- Kerby A, Jones ES, Jones PM, King AJ. Co-transplantation of islets with mesenchymal stem cells in microcapsules demonstrates graft outcome can be improved in an isolated-graft model of islet transplantation in mice. Cytotherapy 2013. 15(2):192-200. [DOD] [CrossRef]
- Veriter S, Gianello P, Igarashi Y, Beaurin G, Ghyselinck A, Aouassar N, Jordan B, Gallez B, Dufrane D. Improvement of subcutaneous bioartificial pancreas vascularization and function by co-encapsulation of pig islets and mesenchymal stem cells in primates. Cell Transplant 2014. 23(11):1349-1364. [DOD] [CrossRef]
- Jourdan G, Dusseault J, Benhamou PY, Rosenberg L, Hallé JP. Co-encapsulation of bioengineered IGF-II-producing cells and pancreatic islets: effect on beta-cell survival. Gene Ther 2011. 18(6):539-545. [DOD] [CrossRef]
- Mathe Z, Bucher P, Bosco D, Andres A, Fux C, Toso C, Oberholzer J, Wandrey C, Sainz-Vidal D, Espinosa D, et al. Short-term immunosuppression reduces fibrotic cellular infiltration around barium-M-alginate microbeads injected intraportally. Transplant Proc 2004. 36(4):1199-1200. [DOD] [CrossRef]
- Hsu BR, Fu SH, Hsueh C, Tsai JS, Huang YY, Huang HS. 15-Deoxyspergualin attenuates pericapsular cellular infiltration and prolongs survival of alginate-poly-L-lysine-alginate microencapsulated islets. Transplant Proc 1997. 29(4):2158-2160. [DOD] [CrossRef]
- Zhang WJ, Marx SK, Laue C, Hyder A, Juergensen A, Bickel M, Schrezenmeir J. HOE 077 reduces fibrotic overgrowth around the barium alginate microcapsules. Transplant Proc 2000. 32(1):206-209. [DOD] [CrossRef]
- Qi M. Transplantation of encapsulated pancreatic islets as a treatment for patients with type 1 diabetes mellitus. Advances in Medicine 2014. In press. [DOD]
- Yang HK, Yoon KH. Current status of encapsulated islet transplantation. J Diabetes Complications 2015. 29(5):737-743. [DOD] [CrossRef]
- Qi M, Strand BL, Mørch Y, Lacík I, Wang Y, Salehi P, Barbaro B, Gangemi A, Kuechle J, Romagnoli T, et al. Encapsulation of human islets in novel inhomogeneous alginate-Ca2+/Ba2+ microbeads: in vitro and in vivo function. Artif Cells Blood Substit Immobil Biotechnol 2008. 36(5):403-420. [DOD] [CrossRef]
- Paredes-Juarez GA, Sahasrabudhe NM, Tjoelker RS, de Haan BJ, Engelse MA, de Koning EJP, Faas MM, de Vos P. DAMP production by human islets under low oxygen and nutrients in the presence or absence of an immunoisolating-capsule and necrostatin-1. Sci Rep 2015. 5:14623. [DOD] [CrossRef]
- Kobayashi T, Aomatsu Y, Iwata H, Kin T, Kanehiro H, Hisanga M, Ko S, Nagao M, Harb G, Nakajima Y. Survival of microencapsulated islets at 400 days post transplantation in the omental pouch of NOD mice. Cell Transplant 2006. 15(4):359-365. [DOD] [CrossRef]
- Dufrane D, Goebbels RM, Saliez A, Guiot Y, Gianello P. Six-month survival of microencapsulated pig islets and alginate biocompatibility in primates: proof of concept. Transplantation 2006. 81(9):1345-1353. [DOD] [CrossRef]
- Caiazzo R, Gmyr V, Hubert T, Delalleau N, Lamberts R, Moerman E, Kerr-Conte J, Pattou F. Evaluation of alternative sites for islet transplantation in the minipig: interest and limits of the gastric submucosa. Transplant Proc 2007. 39(8):2620-2623. [DOD] [CrossRef]
- Komori J, Boone L, DeWard A, Hoppo T, Lagasse E. The mouse lymph node as an ectopic transplantation site for multiple tissues. Nat Biotechnol 2012. 30(10):976-983. [DOD] [CrossRef]
- Christoffersson G, Henriksnäs J, Johansson L, Rolny C, Ahlström H, Caballero-Corbalan J, Ralf Segersvärd, Johan Permert, Olle Korsgren, Per-Ola Carlsson, et al. Clinical and experimental pancreatic islet transplantation to striated muscle: establishment of a vascular system similar to that in native islets. Diabetes 2010. 59(10):2569-2578. [DOD] [CrossRef]
- Stagner JI, Rilo HL, White KK. The pancreas as an islet transplantation site. Confirmation in a syngeneic rodent and canine autotransplant model. JOP 2007. 8(5): 628-636. [DOD]
- Kaufman DB, Morel P, Field MJ, Munn SR, Sutherland DE. Purified canine islet autografts. Functional outcome as influenced by islet number and implantation site. Transplantation 1990. 50(3):385-391. [DOD] [CrossRef]
- Cantarelli E, Melzi R, Mercalli A, Sordi V, Ferrari G, Lederer CW, Mrak E, Rubinacci A, Ponzoni M, Sitia G, et al. Bone marrow as an alternative site for islet transplantation. Blood 2009. 114(20):4566-4574. [DOD] [CrossRef]
- Cantarelli E, Piemonti L. Alternative transplantation sites for pancreatic islet grafts. Curr Diab Rep 2011. 11(5):364-374. [DOD] [CrossRef]
- Vaithilingam V, Evans MD, Rowe A, Bean PA, Tuch BE. Co-encapsulation of Target Effector Cells With Mesenchymal Stem Cells Reduces Pericapsular Fibrosis and Improves Graft Survival in a Xenotransplanted Animal Model. Cell Transplant 2016. 25(7):1299-1317. [DOD] [CrossRef]
- Bloch K, Papismedov E, Yavriyants K, Vorobeychik M, Beer S, Vardi P. Photosynthetic oxygen generator for bioartificial pancreas. Tissue Eng 2006. 12(2):337-344. [DOD] [CrossRef]
- Pedraza E, Coronel MM, Fraker CA, Ricordi C, Stabler CL. Preventing hypoxia-induced cell death in beta cells and islets via hydrolytically activated, oxygen-generating biomaterials. Proc Natl Acad Sci U S A 2012. 109(11):4245-4250. [DOD] [CrossRef]
- Barkai U, Weir GC, Colton CK, Ludwig B, Bornstein SR, Brendel MD, Neufeld T, Bremer C, Leon A, Evron Y, et al. Enhanced oxygen supply improves islet viability in a new bioartificial pancreas. Cell Transplant 2013. 22(8):1463-1476. [DOD] [CrossRef]
- Lo JF, Wang Y, Blake A, Yu G, Harvat TA, Jeon H, Oberholzer J, Eddington DT. Islet preconditioning via multimodal microfluidic modulation of intermittent hypoxia. Anal Chem 2012. 84(4):1987-1993. [DOD] [CrossRef]
- Ma Z, Moruzzi N, Catrina SB, Hals I, Oberholzer J, Grill V, Björklund A. Preconditioning with associated blocking of Ca2+ inflow alleviates hypoxia-induced damage to pancreatic β-cells. PLoS One 2013. 8(7):e67498. [DOD] [CrossRef]
- Vaithilingam V, Oberholzer J, Guillemin GJ, Tuch BE. Beneficial effects of desferrioxamine on encapsulated human islets - in vitro and in vivo study. Am J Transplant 2010. 10(9):1961-1969. [DOD] [CrossRef]
- Moya ML, Garfinkel MR, Liu X, Lucas S, Opara EC, Greisler HP, Brey EM. Fibroblast growth factor-1 (FGF-1) loaded microbeads enhance local capillary neovascularization. J Surg Res 2010. 160(2):208-212. [DOD] [CrossRef]
- McQuilling JP, Arenas-Herrera J, Childers C, Pareta RA, Khanna O, Jiang B, Brey EM, Farney AC, Opara EC. New alginate microcapsule system for angiogenic protein delivery and immunoisolation of islets for transplantation in the rat omentum pouch. Transplant Proc 2011. 43(9):3262-3264. [DOD] [CrossRef]
- Pareta R, McQuilling JP, Sittadjody S, Jenkins R, Bowden S, Orlando G, Farney AC, Brey EM, Opara EC. Long-term function of islets encapsulated in a redesigned alginate microcapsule construct in omentum pouches of immune-competent diabetic rats. Pancreas 2014. 43(4):605-613. [DOD] [CrossRef]
- Chae SY, Kim SW, Bae YH. Effect of cross-linked hemoglobin on functionality and viability of microencapsulated pancreatic islets. Tissue Eng 2002. 8(3):379-394. [DOD] [CrossRef]
- Marchioli G, Luca AD, de Koning E, Engelse M, Van Blitterswijk CA, Karperien M, Van Apeldoorn AA, Moroni L. Hybrid polycaprolactone/alginate scaffolds functionalized with VEGF to promote de novo vessel formation for the transplantation of islets of langerhans. Adv Healthc Mater 2016. 5(13):1606-1616. [DOD] [CrossRef]
- Yin N, Han Y, Xu H, Gao Y, Yi T, Yao J, Dong L, Cheng D, Chen Z. VEGF-conjugated alginate hydrogel prompt angiogenesis and improve pancreatic islet engraftment and function in type 1 diabetes. Mater Sci Eng C Mater Biol Appl 2016. 59:958-964. [DOD]
- Pepper A, Gala-Lopez B, Pawlick R, Merani S, Kin T, Shapiro AM. A prevascularized subcutaneous device-less site for islet and cellular transplantation. Nat Biotechnol 2015. 33(5):518-523. [DOD] [CrossRef]
- Shen F, Li AA, Cornelius RM, Cirone P, Childs RF, Brash JL, Chang PL. Biological properties of photocrosslinked alginate microcapsules. J Biomed Mater Res B Appl Biomater 2005. 75(2):425-434. [DOD] [CrossRef]
- Hill RS, Cruise GM, Hager SR, Lamberti FV, Yu X, Garufis CL, Yu Y, Mundwiler KE, Cole JF, Hubbell JA, et al. Immunoisolation of adult porcine islets for the treatment of diabetes mellitus. The use of photo polymerizable polyethylene glycol in the conformal coating of mass-isolated porcine islets. Ann N Y Acd Sci 1997. 831:332-343. [DOD] [CrossRef]
- Scharp DW, Marchetti P. Encapsulated islets for diabetes therapy: history, current progress, and critical issues requiring solution. Adv Drug Deliv Rev 2014. 67-68:35-73. [DOD] [CrossRef]
- Tomei AA, Manzoli V, Fraker CA, Giraldo J, Velluto D, Najjar M, Pileggi A, Molano RD, Ricordi C, Stabler CL, et al. Device design and materials optimization of conformal coating for islets of Langerhans. Proc Natl Acad Sci U S A 2014. 111(29):10514-10519. [DOD] [CrossRef]
- Giraldo JA, Molano RD, Rengifo HR, Fotino C, Gattás-Asfura KM, Pileggi A, Stabler CL. The impact of cell surface PEGylation and short-course immunotherapy on islet graft survival in an allogeneic murine model. Acta Biomater 2017. 49:272-283. [DOD] [CrossRef]
- Jang JY, Lee DY, Park SJ, Byun Y. Immune reactions of lymphocytes and macrophages against PEG-grafted pancreatic islets. Biomaterials 2004. 25(17):3663-3669. [DOD] [CrossRef]
- Wilson JT, Cui W, Chaikof EL. Layer-by-layer assembly of a conformal nanothin PEG coating for intraportal islet transplantation. Nano Lett 2008. 8(7):1940-1948. [DOD] [CrossRef]
- Krol S, del Guerra S, Grupillo M, Diaspro A, Gliozzi A, Marchetti P. Multilayer nanoencapsulation. New approach for immune protection of human pancreatic islets. Nano Lett 2006. 6(9):1933-1939. [DOD] [CrossRef]
- Golab K, Kizilel S, Bal T, Hara M, Zielinski M, Grose R, Savari O, Wang XJ, Wang LJ, Tibudan M, et al. Improved coating of pancreatic islets with regulatory T cells to create local immunosuppression by using the biotin-polyethylene glycol-succinimidyl valeric acid ester molecule. Transplant Proc 2014. 46(6):1967-1971. [DOD] [CrossRef]
- Kizilel S, Scavone A, Liu X, Nothias JM, Ostrega D, Witkowski P, Millis M. Encapsulation of pancreatic islets within nanothin functional polyethylene glycol coatings for enhanced insulin secretion. Tissue Eng Part A 2010. 16(7):2217-2228. [DOD] [CrossRef]
- Zhi ZL, Kerby A, King AJ, Jones PM, Pickup JC. Nano-scale encapsulation enhances allograft survival and function of islets transplanted in a mouse model of diabetes. Diabetologia 2012. 55(4):1081-1090. [DOD] [CrossRef]
- Dong H, Fahmy TM, Metcalfe SM, Morton SL, Dong X, Inverardi L, Adams DB, Gao W, Wang H. Immuno-isolation of pancreatic islet allografts using pegylated nanotherapy leads to long-term normoglycemia in full MHC mismatch recipient mice. PLoS One 2012. 7(12):e50265. [DOD] [CrossRef]
- Pham-Hua D, Padgett LE, Xue B, Anderson B, Zeiger M, Barra JM, Bethea M, Hunter CS, Kozlovskaya V, Kharlampieva E, et al. Islet encapsulation with polyphenol coatings decreases pro-inflammatory chemokine synthesis and T cell trafficking. Biomaterials 2017. 128:19-32. [DOD] [CrossRef]
- Haque MR, Kim J, Park H, Lee HS, Lee KW, Hilal TA, Jeong JH, Ahn CH, Lee DS, Kim SJ, et al. Xenotransplantation of layer-by-layer encapsulated non-human primate islets with a specified immunosuppressive drug protocol. J Control Release 2017. 258:10-21. [DOD] [CrossRef]
- An D, Ji Y, Chiu A, Lu YC, Song W, Zhai L, Qi L, Luo D, Ma M. Developing robust, hydrogel-based, nanofiber-enabled encapsulation devices (NEEDs) for cell therapies. Biomaterials 2015. 37:40-48. [DOD] [CrossRef]
- Nyitray CE, Chang R, Faleo G, Lance KD, Bernards DA, Tang Q, Desai TA. Polycaprolactone Thin-Film Micro- and Nanoporous Cell-Encapsulation Devices. ACS Nano 2015. 9(6):5675-5682. [DOD] [CrossRef]
- Sabek OM, Ferrati S, Fraga DW, Sih J, Zabre EV, Fine DH, Ferrari M, Gaber AO, Grattoni A. Characterization of a nanogland for the autotransplantation of human pancreatic islets. Lab Chip 2013. 13(18):3675-3688. [DOD] [CrossRef]
- Harrington S, Williams J, Rawal S, Ramachandran K, Stehno-Bittel L. Hyaluronic acid/collagen hydrogel as an alternative to alginate for long-term immunoprotected islet transplantation. Tissue Eng Part A 2017. In press. [DOD]
- Sharma V, Hunckler M, Ramasubramanian MK, Opara EC, Katuri KC. Microfluidic Approach to Cell Microencapsulation. Methods Mol Biol 2017. 1479:71-76. [DOD] [CrossRef]
- de Vos P, Wolters GHJ, van Schilfgaarde R. Possible relationship between fibrotic overgrowth of alginate-polylysine- alginate microencapsulated pancreatic islets and the microcapsule integrity. Transplant Proc 1994. 26(2):782-783. [DOD]
- de Vos P, Faas MM, Strand B, Calafiore R. Alginate-based microcapsules for immunoisolation of pancreatic islets. Biomaterials 2006. 27(32):5603-5617. [DOD] [CrossRef]
- Horcher A, Zekorn T, Siebers U, Klöck G, Frank H, Houben R, Bretzel RG, Zimmermann U, Federlin K. Transplantation of microencapsulated islets in rats: evidence for induction of fibrotic overgrowth by islet alloantigens released from microcapsules. Transplant Proc 1994. 26(2):784-786. [DOD]
- Grimm J, Kircher MF, Weissleder R. Cell tracking. Radiologe 2007. 47(1):25-33. [DOD] [CrossRef]
- Long CM, Bulte JWM. In vivo tracking of cellular therapeutics using magnetic resonance imaging. Expert Opin Biol Ther 2009. 9(3):293-306. [DOD] [CrossRef]
- Barnett BP, Arepally A, Karmarkar PV, Qian D, Gilson WD, Walczak P, Howland V, Lawler L, Lauzon C, Stuber M, et al. Magnetic resonance-guided, real-time targeted delivery and imaging of magnetocapsules immunoprotecting pancreatic islet cells. Nat Med 2007. 13(8):986-991. [DOD] [CrossRef]
- Shapiro EM, Skrtic S, Sharer K, Hill JM, Dunbar CE, Koretsky AP. MRI detection of single particles for cellular imaging. Proc Natl Acad Sci U S A 2004. 101(30):10901-10906. [DOD] [CrossRef]
- Vaithilingam V, Yim MM, Foster JL, Stait-Gardner T, Oberholzer J, Tuch BE. Non-invasive tracking of encapsulated insulin producing cells labelled with magnetic microspheres by magnetic resonance imaging. J Diabetes Res 2016. 2016:6165893. [DOD] [CrossRef]
- Barnett BP, Ruiz-Cabello J, Hota P, Liddell R, Walczak P, Howland V, Chacko VP, Kraitchman DL, Arepally A, Bulte JW. Fluorocapsules for improved function, immunoprotection, and visualization of cellular therapeutics with MR, US, and CT imaging. Radiology 2011. 258(1):182-191. [DOD] [CrossRef]
- Arifin DR, Long CM, Gilad AA, Alric C, Roux S, Tillement O, Link TW, Arepally A, Bulte JW. Trimodal gadolinium-gold microcapsules containing pancreatic islet cells restore normoglycemia in diabetic mice and can be tracked by using US, CT, and positive-contrast MR imaging. Radiology 2011. 260(3):790-798. [DOD] [CrossRef]
- Barnett BP, Kraitchman DL, Lauzon C, Magee CA, Walczak P, Gilson WD, Arepally A, Bulte JW. Radiopaque alginate microcapsules for X-ray visualization and immunoprotection of cellular therapeutics. Mol Pharm 2006. 3(5):531-538. [DOD] [CrossRef]
- Astolfo A, Qie F, Kibleur A, Hao X, Menk RH, Arfelli F, Rigon L, Hinton TM, Wickramaratna M, Tan T, et al. A simple way to track single gold-loaded alginate microcapsules using x-ray CT in small animal longitudinal studies. Nanomedicine 2014. 10(8):1821-1828. [DOD] [CrossRef]
- Qie F, Astolfo A, Wickramaratna M, Behe M, Evans MD, Hughes TC, Hao X, Tan T. Self-assembled gold coating enhances X-ray imaging of alginate microcapsules. Nanoscale 2015. 7(6):2480-2488. [DOD] [CrossRef]
- Korbutt GS, Elliott JF, Ao Z, Smith DK, Warnock GL, Rajotte RV. Large scale isolation, growth, and function of porcine neonatal islet cells. J Clin Invest 1996. 97(9):2119-2129. [DOD] [CrossRef]
- Pagliuca FW, Millman JR, Gurtler M, Segel M, Van Dervort A, Ryu JH, Peterson QP, Greiner D, Melton DA. Generation of functional human pancreatic beta cells in vitro. Cell 2014. 159(2):428-439. [DOD] [CrossRef]
- Rezania A, Bruin JE, Arora P, Rubin A, Batushansky I, Asadi A, O'Dwyer S, Quiskamp N, Mojibian M, Albrecht T, et al. Reversal of diabetes with insulin-producing cells derived in vitro from human pluripotent stem cells. Nat Biotechnol 2014. 32(11):1121-1133. [DOD] [CrossRef]
- Millman JR, Xie C, Van Dervort A, Gürtler M, Pagliuca FW, Melton DA. Generation of stem cell-derived beta-cells from patients with type 1 diabetes. Nat Commun 2016. 7:11463. [DOD] [CrossRef]
- Chayosumrit M, Tuch B, Sidhu K. Alginate microcapsule for propagation and directed differentiation of hESCs to definitive endoderm. Biomaterials 2010. 31(3):505-514. [DOD] [CrossRef]
- Richardson T, Kumta PN, Banerjee I. Alginate encapsulation of human embryonic stem cells to enhance directed differentiation to pancreatic islet-like cells. Tissue Eng Part A 2014. 20(23-24):3198-3211. [DOD] [CrossRef]
- Richardson T, Barner S, Candiello J, Kumta PN, Banerjee I. Capsule stiffness regulates the efficiency of pancreatic differentiation of human embryonic stem cells. Acta Biomater 2016. 35:153-165. [DOD] [CrossRef]
- Agulnick AD, Ambruzs DM, Moorman MA, Bhoumik A, Cesario RM, Payne JK, Kelly JR, Haakmeester C, Srijemac R, Wilson AZ, et al. Insulin-producing endocrine cells differentiated in vitro from human embryonic stem cells function in macroencapsulation devices in vivo. Stem Cells Transl Med 2015. 4(10):1214-1222. [DOD] [CrossRef]
- Kroon E, Martinson LA, Kadoya K, Bang AG, Kelly OG, Eliazer S, Young H, Richardson M, Smart NG, Cunningham J, et al. Pancreatic endoderm derived from human embryonic stem cells generates glucose-responsive insulin-secreting cells in vivo. Nat Biotechnol 2008. 26(4):443-452. [DOD] [CrossRef]
- Rezania A, Xu J, Narayan K, Fox JK, O'Neil JJ, Kieffer TJ, Bruin JE. Maturation and function of human embryonic stem cell-derived pancreatic progenitors in macroencapsulation devices following transplant into mice. Diabetologia 2013. 56(9):1987-1998. [DOD] [CrossRef]
- Kirk K, Hao E, Lahmy R, Itkin-Ansari P. Human embryonic stem cell derived islet progenitors mature inside an encapsulation device without evidence of increased biomass or cell escape. Stem Cell Res 2014. 12(3):807-814. [DOD] [CrossRef]
- Boettler T, Schneider D, Cheng Y, Kadoya K, Brandon EP, Martinson L, von Herrath M. Pancreatic tissue transplanted in theracyte encapsulation devices is protected and prevents hyperglycemia in a mouse model of immune-mediated diabetes. Cell Transplant 2016. 25(3):609-614. [DOD] [CrossRef]
- Yakhnenko I, Wong WK, Katkov II, Itkin-Ansari P. Cryopreservation of human insulin expressing cells macro-encapsulated in a durable therapeutic immunoisolating device theracyte. Cryo Letters 2012. 33(6):518-531. [DOD]
- Chun SY, Mack DL, Moorefield E, Oh SH, Kwon TG, Pettenati MJ, Yoo JJ, Coppi PD, Atala A, Soker S. Pdx1 and controlled culture conditions induced differentiation of human amniotic fluid-derived stem cells to insulin-producing clusters. J Tissue Eng Regen Med 2015. 9(5):540-549. [DOD] [CrossRef]
- Chandra V, Swetha G, Muthyala S, Jaiswal AK, Bellare JR, Nair PD, Bhonde RR. Islet-like cell aggregates generated from human adipose tissue derived stem cells ameliorate experimental diabetes in mice. PLoS One 2011. 6(6):e20615. [DOD] [CrossRef]
- Davis NE, Beenken-Rothkopf LN, Mirsoian A, Kojic N, Kaplan DL, Barron AE, Fontaine MJ. Enhanced function of pancreatic islets co-encapsulated with ECM proteins and mesenchymal stromal cells in a silk hydrogel. Biomaterials 2012. 33(28):6691-6697. [DOD] [CrossRef]
- Arlov O, Aachmann FL, Feyzi E, Sundan A, Skjåk-Bræk G. The impact of chain length and flexibility in the interaction between sulfated alginates and HGF and FGF-2. Biomacromolecules 2015. 16(11):3417-3424. [DOD] [CrossRef]
- Chen X, Shao W, Chen JB, Zhang L, Matthias C, Shan SG, Qi ZQ. Allotransplantation of sulphate glucomannan-alginate barium (SGA)-microencapsulated rat islets for the treatment of diabetes mellitus. Immunol Invest 2009. 38(7):561-571. [DOD] [CrossRef]
- Dufrane D, Steenberghe Mv, Goebbels RM, Saliez A, Guiot Y, Gianello P. The influence of implantation site on the biocompatibility and survival of alginate encapsulated pig islets in rats. Biomaterials 2006. 27(17):3201-3208. [DOD] [CrossRef]
- Arifin DR, Valdeig S, Anders RA, Bulte JW, Weiss CR. Magnetoencapsulated human islets xenotransplanted into swine: a comparison of different transplantation sites. Xenotransplantation 2016. 23(3):211-221. [DOD] [CrossRef]
- Pareta R, McQuilling JP, Sittadjody S, Jenkins R, Bowden S, Orlando G, Farney AC, Brey EM, Opara EC. Long-term function of islets encapsulated in a redesigned alginate microcapsule construct in omentum pouches of immune-competent diabetic rats. Pancreas 2014. 43(4):605-613. [DOD] [CrossRef]
|