Original Data
Rev Diabet Stud,
2019,
15:83-93 |
DOI 10.1900/RDS.2019.15.83 |
The Combination of Whey Protein and Dietary Fiber Does Not Alter Low-Grade Inflammation or Adipose Tissue Gene Expression in Adults with Abdominal Obesity
Elin Rakvaag1, Rasmus Fuglsang-Nielsen1, Knud Erik Bach Knudsen2, Kjeld Hermansen1,3, Søren Gregersen1,4
1Department of Endocrinology and Internal Medicine, Aarhus University Hospital, 8200 Aarhus N, Denmark
2Department of Animal Science, Aarhus University, 8830 Tjele, Denmark
3Department of Clinical Medicine, Aarhus University, 8200 Aarhus N, Denmark
4Steno Diabetes Center Aarhus, 8200 Aarhus N, Denmark
Address correspondence to: Elin Rakvaag, e-mail: elinrak1210@gmail.com
Manuscript submitted October 18, 2019; resubmitted December 4, 2019; accepted December 9, 2019; published in print December 30, 2019.
Keywords: whey protein, wheat bran, dietary fiber, inflammation, adipose tissue gene expression
Abstract
BACKGROUND: Abdominal obesity is characterized by low-grade inflammation and plays a central role in the development of type 2 diabetes and cardiovascular diseases. Dietary factors can influence low-grade inflammation and affect adipose tissue function. AIM: To investigate the separate and combined effects of whey protein and cereal fiber on inflammatory markers and adipose tissue gene expression in abdominal obesity. METHODS: We performed a 12-week, double-blind, randomized controlled dietary intervention in 65 adults with abdominal obesity. The participants were randomized to 4 groups using a 2 × 2 factorial design; they received either 60 g/day of whey protein or maltodextrin in combination with high-fiber wheat bran products (30 g fiber/day) or low-fiber refined wheat products (10 g fiber/day). Plasma concentrations of tumor necrosis factor α (TNF-α), high-sensitivity C-reactive protein (hs-CRP), monocyte chemoattractant protein-1 (MCP-1), interleukin 1 receptor antagonist (IL-1Ra), and adiponectin were measured before and after intervention. Changes in gene expression related to inflammation, insulin signaling, and lipid metabolism were measured in abdominal subcutaneous adipose tissue. RESULTS: After intervention, TNF-α was reduced for both high-fiber groups compared with baseline, but did not significantly differ from the low-fiber groups. There were no differences in fasting or postprandial inflammatory markers between the groups. The relative gene expression of ribosomal protein S6 kinase B1 (S6K1) was increased after whey protein compared with maltodextrin consumption. CONCLUSION: Intake of whey protein in combination with high cereal fiber content did not differentially affect low-grade inflammation or adipose tissue gene expression compared with maltodextrin and low fiber content in individuals with abdominal obesity.
Abbreviations: ACTB - β-actin; AXOS - arabinoxylan oligosaccharides; BCAA - branched-chain amino acid; CCL-5 - CC chemokine ligand-5; CVD - cardiovascular diseases; FDR - false discovery rate ; HiFi - high-fiber intervention; hs-CRP - high-sensitivity C-reactive protein; IL-1Ra - interleukin-1 receptor antagonist; IRS1 - insulin receptor substrate 1 ; LoFi - low-fiber intervention; LPS - lipopolysaccharide; MCP-1 - monocyte chemoattractant protein-1; MD - maltodextrin; MTOR - mammalian target of rapamycin; S6K1 - ribosomal protein S6 kinase B1; SAT - subcutaneous adipose tissue; T2D - type 2 diabetes; TNF-α - tumor necrosis factor-alpha; VAT - visceral adipose tissue; WP - whey protein
1. Introduction
Low-grade inflammation is closely related to obesity and plays a central role in the development of type 2 diabetes (T2D) and cardiovascular diseases (CVD) [1-4]. A number of circulating pro-inflammatory markers are found to be elevated in abdominal obesity, e.g. C-reactive protein (CRP), monocyte chemoattractant protein-1 (MCP-1), and tumor necrosis factor-alpha (TNF-α) [5-7]. Furthermore, postprandial inflammation is exag-gerated in individuals with obesity, and may be involved in the development of insulin resistance, which plays a major role in the development of both T2D and CVD [8-10].
A dietary pattern characterized by low-fat dairy and whole-grain products is positively associated with the anti-inflammatory marker adiponectin [11], and high intake of dairy products is associated with lower levels of CRP and TNF-α [12]. Ob-servational studies indicate that foods rich in cereal fiber, such as whole grains and bran, are associated with lower levels of pro-inflammatory markers [13, 14]. However, results from intervention studies are inconsistent [15]. Randomized, controlled trials have indicated a reduction in CRP concentration following consumption of whey protein (WP) supplements (≥20 g/day) [16]. Concordantly, both milk peptides and dietary fiber may have beneficial effects on low-grade inflammation [17]. However, little is known about the impact of the combination of milk proteins and dietary fiber.
Adipose tissue is an active endocrine organ that releases pro-inflammatory adipokines, including TNF-α, MCP-1, and interleukin-1 receptor antago-nist (IL-1Ra), as well as the anti-inflammatory protein adiponectin. Adipose tissue dysfunction leading to disturbances in the production or secre-tion of adipokines may increase insulin resistance in abdominal obesity [18]. Dietary constituents have also been found to affect gene expression in adipose tissue; high-protein diets have been shown to downregulate subcutaneous adipose tissue (SAT) expression of genes involved in lipid metabolism [19], while a high-fiber/low-insulin-response diet has been shown to downregulate genes involved in insulin signaling [20].
In this article, we aimed to investigate the ef-fects of WP supplements and high-fiber diets on circulating inflammatory markers (both fasting and postprandial) and to analyze SAT expression of genes involved in inflammation, lipid metabolism, and insulin signaling. We hypothesized that intake of WP and high-fiber cereal products (either in combination or separately) would have beneficial effects on inflammation compared with isoenergetic intake of maltodextrin (MD) and low-fiber cereal products. This was tested in a 12-week, randomized controlled, double-blind dietary intervention study in adults with abdominal obesity.
2. Methods
The present study is part of the MERITS study that investigates the effects of WP and cereal fiber on lipemia and metabolic changes. The study was conducted at the Department of Endocrinology and Internal Medicine, Aarhus University Hospital, Denmark, between May 2016 and June 2017. The study protocol was approved by the Central Denmark Region Committees on Health Research Ethics (Journal no. 1-10-72-370-15). Details of study design, participants, and dietary interventions have been published elsewhere [21].
2.1 Study design, participants, and dietary intervention
We conducted a double-blind, randomized controlled, parallel intervention with a 2 × 2 factorial design. Inclusion and exclusion criteria have been described in detail elsewhere [21]. In short, 73 men and women (age ≥40 years) with abdominal obesity (waist circumference ≥80 cm for women and ≥94 cm for men) were randomized to 1 of 4 intervention diets for 12 weeks preceded by one week of run-in.
The participants were provided with isocaloric powder supplements containing either 2 × 30 g/d WP (Lacprodan® HYDRO.REBUILD) or MD (Glucidex® 19), in combination with either wheat bran products containing 30 g/d dietary fiber (HiFi) or refined wheat products containing 10 g/d dietary fiber (LoFi). The powder supplements were provided by Arla Foods Ingredients Group P/S (Viby, Denmark) and the wheat bran by Lantmännen Cerealia AB (Malmø, Sweden). The wheat bran was treated with cell wall-degrading enzymes (xylanase, glucanase, cellulase) by DuPont Industrial Biosciences Aps (Brabrand, Denmark) in order to increase the content of arabinoxylan oligosaccharides (AXOS) and improve the baking properties of the bran [22].
The participants were instructed to replace their habitual intake of bread and cereal products with the products provided, and to consume the powder supplements twice daily. They were encouraged not to change other dietary habits during the intervention period and to maintain their physical activity level to ensure weight stability. Both participants and study personnel remained blinded to the group allocations throughout the study. Compliance was assessed by measuring plasma alkylresorcinols (a marker of cereal bran intake) and urinary carbamide excretion (a protein intake marker). Also, the participants filled out a daily test product journal that was used to monitor compliance during the trial.
Before and after the 12-week intervention, the participants underwent a standardized, high-fat meal test (4700 kJ, 70 g of fat) following an overnight fast, as described previously [21]. A catheter was placed in an antecubital vein for blood sampling. Fasting blood samples were collected for measurement of TNF-α, IL-1Ra, hs-CRP, MCP-1, and adiponectin. During the test meal, postprandial blood samples were collected at t = 30, 60, 120, and 240 min for measurement of MCP-1, and at t = 30, 60, 120, and 360 for measurement of adiponectin.
2.2 Measurement of inflammatory markers
Blood samples for measuring TNF-α, IL-1Ra, and adiponectin were immediately centrifuged at 2000 × g for 15 min at 4 °C. Serum samples for measuring MCP-1 and hs-CRP were left at room temperature for 30 min before being centrifuged for 10 min at 2000 × g. Plasma and serum samples were then frozen at -20 °C and moved to -80 °C within 8 h for storage.
TNF-α, MCP-1, IL-1Ra, and adiponectin were all measured using ELISA technique. TNF-α was determined using a human Quantikine® High Sensitivity kit (cat. HSTA00E, R&D Systems, Minneapolis, USA), with assay range of 0.2-10 pg/ml, intra-assay precision of 1.9-2.2%, and inter-assay precision of 6.2-6.7%. MCP-1 was measured using LEGEND MAX™ Human MCP-1/CCL2 ELISA kit with pre-coated plates (cat. 438808, BioLegend, San Diego, USA), with assay range of 7.8-500 pg/ml, intra-assay precision of 6.0-6.4%, and inter-assay precision of 1.8-6.0%. Adiponectin was determined using a human adiponectin ELISA kit (cat. K1001-1, B-Bridge International, Inc., Santa Clara, USA), with assay range 0.375-12.0 ng/ml, intra-assay precision 4.6-5.8%, and inter-assay precision 3.2-7.3%. IL-1Ra was determined using a human Quantikine® kit (cat. DRA00B, R&D Systems, Minneapolis, USA), with assay range of 31.2-2000 pg/ml, intra-assay precision of 3.7-7.3%, and inter-assay precision of 6.7-11.0%. Hs-CRP was measured on a Cobas c 111 system using a commercial kit (ref. 05401607, Roche Diagnostics GmbH, Mannheim), with assay range of 0.15-20.0 mg/l, intra-assay precision of 0.3-1.5%, and inter-assay precision of 0.7-2.0%.
2.3 Adipose tissue biopsies
Biopsies were collected from abdominal SAT before and after dietary intervention. The procedure was performed under local analgesia using a Bergström needle. The tissue biopsies were cleaned with saline and snap-frozen in liquid nitrogen before storage at -80 °C.
2.4 Gene expression analyses
RNA purification and gene expression analyses were performed by BioXpedia A/S (Aarhus, Denmark), using real-time qPCR with pre-designed primers and TaqMan gene expression assays (Applied Biosystems, Life Technologies, California, USA). An overview of the assay identification numbers is given in supplementary Table A1. We measured the cycle threshold (CT) values for each sample in triplicate. The mean fold change in the target genes, normalized to the reference gene β-actin (ACTB) and relative to the expression at week 0, was calculated by the 2−ΔΔCT method [23]. The expression of the reference gene ACTB was not different between groups at baseline, and was not affected by the different dietary interventions (assessed by one-way ANOVA).
2.5 Calculations and statistical analyses
The postprandial responses in MCP-1 and adiponectin before and after intervention were calculated as total areas under the curve (AUC) for 240 min (MCP-1) and 360 min (adiponectin) using the trapezoidal rule.
Statistical analyses were performed in Stata IC/15.1 (StataCorp LP College Station, TX, USA), and graphical elements were constructed in GraphPad Prism 7.04 (Graphpad Software, CA, USA). P-values < 0.05 were considered statistically significant. Variables were assessed for normal distribution by quantile-quantile plots and histograms, and homogeneity of variance was assessed by Bartlett's test. The distribution of paired data was further assessed by Bland-Altman plots. If the data were found not to be normally distributed, log-transformation was applied and the data were reassessed.
We tested between-group differences in inflammatory markers using a nonparametric Kruskal-Wallis test, and within-group changes in inflammatory markers from baseline using Wilcoxon signed-rank test because data did not fulfill criteria for normal distribution and/or homogeneity of variance after log-transformation. Differences in relative gene expression between diets were assessed by a two-factor ANOVA adjusted for age and sex. Pairwise comparisons of groups were corrected for multiple comparisons by the Tukey-Kramer method. Data were checked by diagnostic plots of residuals (quantile-quantile plots, histograms, and residuals versus fitted plots). We assessed within-group changes in relative gene expression from baseline by paired t-tests. We applied the Benjamini-Hochberg procedure with a false discovery rate (FDR) of 0.25 to account for multiple statistical testing of the gene expression data [24].
3. Results
In total, 65 participants completed the study. Samples for fasting circulating inflammatory markers were obtained from all participants (34 women and 31 men). Data on postprandial MCP-1 at baseline are missing for two participants because of blood sampling issues. Adipose tissue biopsies were collected from 61 participants (32 women and 29 men); one participant refused biopsy and three were excluded from biopsies because of anticoagulant medication use. We have described the baseline participant characteristics previously [21]. Briefly, the study population had a median age of 64 years (range 40-75), a mean BMI of 29.4 ± 3.7 kg/m2, and 52% fulfilled the International Diabetes Federation criteria for the metabolic syndrome. The participants remained weight stable throughout the intervention [21].
3.1 Inflammation
Fasting inflammatory markers at baseline and week 12 are presented in Table 1. After intervention, we observed a decrease in fasting TNF-α for the two HiFi groups, but this change did not significantly differ from the LoFi groups. Compared with baseline, TNF-α was decreased by 12% for WP-HiFi (95% CI: 4-19%, p < 0.05), and by 9% for MD-HiFi (95% CI: 2-16%, p < 0.05). Furthermore, we found a 13% decrease in fasting IL-1Ra for MD-LoFi after intervention (95% CI: 2-25%, p < 0.05); however, this was not significantly different from the other groups.
Table
1.
Fasting inflammatory markers before and after the 12-week intervention |
|
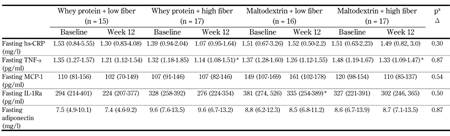 |
 |
Legend:
Values are median and centiles (25th-75th). a P-values for differences in Δ (week 12 - baseline) between diet groups, assessed by nonparametric Kruskal-Wallis test. * Significantly different from baseline (p < 0.05) assessed by Wilcoxon signed-rank test. Abbreviations: hs-CRP - high-sensitivity C-reactive protein, IL-1Ra - interleukin 1 receptor antagonist, MCP-1 - monocyte chemoattractant protein-1, TNF-α - tumor necrosis factor α. |
|
We found no changes in fasting hs-CRP, MCP-1, or adiponectin for either of the intervention groups. Furthermore, we observed no changes in postprandial responses of MCP-1 or adiponectin following the interventions (Table 2). MCP-1 increased after high-fat meals both at baseline and week 12, and peaked 120 min postprandially for all groups. For adiponectin, there was a small initial increase 30-60 min postprandially for all groups (data not shown).
Table
2.
Postprandial response for inflammatory markers (AUC) during a high-fat meal test at baseline (week 0) and week 12 |
|
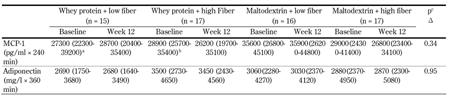 |
 |
Legend:
Values are medians and centiles (25th-75th). a n = 14. b n = 16. c P-value for differences in Δ (week 12 - baseline) between diet groups, assessed by nonparametric Kruskal-Wallis test. Abbreviations: AUC - area under the curve, hs-CRP - high-sensitivity C-reactive protein, IL-1Ra - interleukin-1 receptor antagonist, MCP-1 - monocyte chemoattractant protein-1. |
|
3.2 Gene expression
Relative changes in SAT gene expression after the 12-week intervention period are shown in Figures 1-3. There was a main effect of protein level on ribosomal protein S6 kinase B1 (S6K1) gene expression (p = 0.02), showing an increase after WP consumption compared with MD consumption. After intervention, the relative gene expression of S6K1 was significantly increased for WP-HiFi compared with MD-HiFi (p = 0.02 after correction for multiple comparisons) (Figure 3). MD-HiFi had a greater increase in resistin (RETN) gene expression compared with MD-LoFi (p = 0.01 after correction for multiple comparisons) (Figure 1). Any within-group changes in gene expression from baseline were not statistically significant after FDR correction.
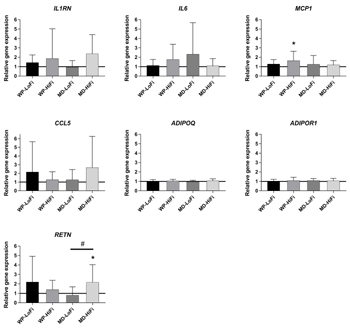 |
 |
Figure 1. Relative changes from baseline to week 12 in the expression of selected genes involved in inflammation (mean and SD). Gene expression was measured in subcutaneous adipose tissue of 61 participants (WP-LoFi: n = 15, WP-HiFi: n = 16, MD-LoFi: n = 14, MD-HiFi: n = 16), except for RETN, where N = 59 due to measurement error (WP-LoFi: n = 14, WP-HiFi: n = 16, MD-LoFi: n = 14, MD-HiFi: n = 15). # Significant difference between groups assessed by two-factor ANOVA (p < 0.05 after correction for multiple comparisons by the Tukey-Kramer method). * Within-group change from baseline assessed by paired t-test (p < 0.05 before FDR correction, p > 0.05 after FDR correction). Abbreviations: FDR – false discovery rate, MD-HiFi – maltodextrin + high fiber, MD-LoFi – maltodextrin + low fiber, WP-HiFi – whey protein + high fiber, WP-LoFi – whey protein + low fiber. |
|
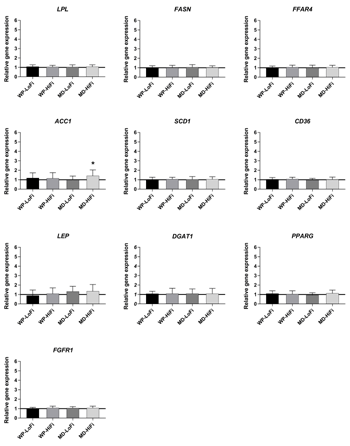 |
 |
Figure 2. Relative changes from baseline to week 12 in the expression of selected genes involved in lipid metabolism and cell differentiation (mean and SD). Gene expression was measured in subcutaneous adipose tissue of 61 participants (WP-LoFi: n = 15, WP-HiFi: n = 16, MD-LoFi: n = 14, MD-HiFi: n = 16). * Within-group change from baseline assessed by paired t-test (p < 0.05 before FDR correction, p > 0.05 after FDR correction). Abbreviations: FDR – false discovery rate, MD-HiFi – maltodextrin + high fiber, MD-LoFi – maltodextrin + low fiber, WP-HiFi – whey protein + high fiber, WP-LoFi – whey protein + low fiber. |
|
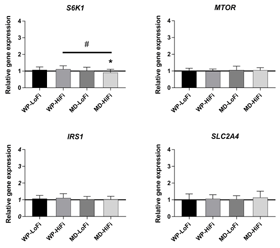 |
 |
Figure 3. Relative changes from baseline to week 12 in the expression of selected genes involved in insulin signaling (mean and SD). Gene expression was measured in subcutaneous adipose tissue of 61 participants (WP-LoFi: n = 15, WP-HiFi: n = 16, MD-LoFi: n = 14, MD-HiFi: n = 16). # Significant difference between groups assessed by two-factor ANOVA (p < 0.05 after correction for multiple comparisons by the Tukey-Kramer method). * Within-group change from baseline assessed by paired t-test (p < 0.05 before FDR correction, p > 0.05 after FDR correction). Abbreviations: FDR – false discovery rate, MD-HiFi – maltodextrin + high fiber, MD-LoFi – maltodextrin + low fiber, WP-HiFi – whey protein + high fiber, WP-LoFi – whey protein + low fiber. |
|
4. Discussion
In the present study, we investigated the effects of WP supplements or a carbohydrate control in combination with high-fiber or low-fiber cereal products on circulating inflammatory markers and SAT gene expression. We found a reduction in the pro-inflammatory cytokine TNF-α compared with baseline after consumption of the wheat bran-rich diet for 12 weeks. However, this change was not significantly different from that observed in the refined wheat groups.
The MERITS study was originally powered to detect differences in postprandial triglycerides, which was the primary outcome [21]. We cannot exclude that the sample size may have been too small to detect differential effects on plasma cytokines. However, previous studies of similar or smaller sample sizes and shorter durations have detected differences in circulating TNF-α between a wholegrain wheat diet and a refined wheat diet in subjects with overweight and obesity [25, 26]. This discrepancy in results could be due to differences in study populations; the previous studies included only individuals with low intakes of wholegrain and dietary fiber at baseline, while our study population was adapted to a relatively high dietary fiber intake, corresponding to the Danish national average of 22 g/day [27]. It is possible that we would have observed a significant impact of our interventions in individuals with a less healthy background diet.
We found no effects of the interventions on postprandial responses of MCP-1 or adiponectin. A previous study in adults with overweight and obesity found that consumption of wholegrain wheat for 12 weeks reduced the postprandial response of several inflammatory markers compared with consumption of refined wheat [28]; however, they investigated different inflammatory markers than we did in the present study.
While consumption of wholegrain and cereal bran products has been associated with lower levels of inflammation [13, 14, 29], the mechanisms behind this association remain largely unknown, and may be attributed to a range of constituents present in the grain [30, 31]. Wheat grain contains a number of phytochemicals and dietary fiber types, which are mainly located in the bran fraction [32]. In the present study, we treated the wheat bran with cell wall-degrading enzymes prior to incorporating it into the test products. This bioprocessing may potentially have enhanced the availability of bioactive compounds [32]. Interestingly, a previous study showed that bioprocessing of wheat bran with cell wall-degrading enzymes and yeast fermentation greatly increased the bioavailability of phenolic acids, which are considered to possess anti-inflammatory properties [33]. In the same study, bioprocessed wheat bran exerted anti-inflammatory effects in lipopolysaccharide (LPS) stimulated blood ex vivo compared with native wheat bran. In the present study, the enzymatic treatment of the wheat bran changed the composition of the soluble and insoluble dietary fiber fractions, particularly increasing the AXOS content. Enzyme-treated wheat bran with an increased AXOS content has been shown to induce anti-inflammatory effects in LPS-stimulated mice [34], but this effect is yet to be demonstrated in low-grade inflammation.
Except for the within-group reduction in TNF-α in the group receiving WP-HiFi, we found no effects of the WP intervention on low-grade inflammation. A study conducted in patients with acute ischemic stroke showed beneficial effects on inflammation after short-term enteral feeding with WP [35]. However, results from trials in healthier study populations, using a similar duration and WP dose to those in the present study, corroborate our results [36-38].
Furthermore, we found no long-term differences in postprandial responses of MCP-1 or adiponectin between the interventions. To our knowledge, few previous studies have investigated the long-term effects of dietary interventions on fat-induced postprandial inflammation. A study from our group previously reported no effects on postprandial MCP-1 after intake of WP or casein for 12 weeks [37]. However, an acute study detected a higher postprandial MCP-1 response following a high-fat meal with WP compared with other proteins, while the acute response in CC chemokine ligand-5 (CCL5/RANTES) was lower after the WP meal [39]. Another acute study found a reduction in postprandial IL-6 after intake of a fiber-rich wheat-bran meal compared with a potato meal [8]. However, we did not measure circulating CCL5/RANTES or IL-6 in the present study.
We observed a within-group reduction in IL-1Ra in the MD-LoFi group. Circulating IL-1Ra is elevated in obesity [40], and increased levels precede the onset of T2D [41]. A reduction in IL-1Ra after the intervention with MD and low-fiber products could therefore indicate an unexpected beneficial change in this group. In contrast, a control diet with low-fiber cereal products has previously been shown to increase IL-1Ra compared with a healthy, high-fiber Nordic diet [42]. In this regard, it is relevant to point out that the observed reduction from baseline for MD-LoFi was not significantly different compared with the other intervention groups in the present study.
Interestingly, we found an increase in SAT gene expression of S6K1 after WP compared with MD consumption. S6K1 encodes a kinase involved in the mammalian target of rapamycin (MTOR) signaling pathway, a key regulator of protein synthesis [43]. Intake of leucine―a branched-chain amino acid (BCAA) which is abundant in WP―induces activation of S6K1 through phosphorylation [44]. However, it is important to note that we did not measure phosphorylation of S6K1 in the present study. Activation of the MTOR pathway by BCAAs is thought to block insulin signaling via inactivation of insulin receptor substrate 1 (IRS1) [45, 46]. Furthermore, elevated plasma levels of BCAAs are strongly associated with insulin resistance and predict the onset of T2D [47, 48]. Consequently, it has been hypothesized that high-protein diets play a role in the development of insulin resistance [49, 50]. Interestingly, one study found a tendency for higher S6K1 protein expression following 6 weeks of high-protein diet, which was associated with reduced insulin sensitivity [51]. However, these effects were attenuated after 18 weeks. We found no changes in MTOR or IRS1 expression, in accordance with a previous study comparing two different high-protein diets [19]. It would have been interesting to determine the activation of the MTOR pathway in skeletal muscle tissue, since it has been demonstrated in humans that phosphorylation of both MTOR and S6K1 is increased in skeletal muscle following intake of a dairy protein-rich meal [52].
Furthermore, we found an increase in RETN gene expression in SAT after 12 weeks of MD-HiFi compared with MD-LoFi. RETN encodes resistin, a protein with pro-inflammatory properties which has been linked to abdominal obesity and insulin resistance. The role of resistin is, however, still inconclusive [53]. The increased RETN gene expression in MD-HiFi could indicate an unfavorable change in inflammation in this group. However, this finding should be interpreted with caution, since we found no other indications of increased inflammation in this group. We observed no differences between interventions in the expression of genes related to lipid metabolism, despite our previously reported findings regarding beneficial changes in the plasma lipid profile after the WP-LoFi diet [21].
A limitation of our study is that for most of the markers of inflammation, insulin signaling, and lipid metabolism we measured mRNA levels only; changes in gene expression do not necessarily correspond to changes in plasma protein levels [54]. Furthermore, we observed a large variation in mRNA levels of several of the genes related to inflammation. It is possible that some of this variability is due to viral infections during the trial, although the few cases of self-reported illnesses among our participants do not explain the outliers (data not shown). It would have been interesting to measure gene expression in visceral adipose tissue (VAT) also, since studies comparing SAT and VAT have detected depot-specific differences in gene expression, with VAT showing higher expression of certain genes involved in inflammation and lipid metabolism [55].
An important strength of our study is that the participants were weight stable during the intervention. Weight loss has great influence on low-grade inflammation [17], and differences in weight change between groups would have made it difficult to evaluate the effect of the test products themselves. Other strengths of our study include the use of a double-blind, randomized controlled design, and the fact that we measured biochemical markers in addition to self-reported data to verify a high degree of compliance with our interventions. However, the sample size may have been too small to detect differences in inflammatory markers.
In conclusion, intake of WP supplements and high-fiber wheat bran products for 12 weeks did not significantly influence fasting or postprandial markers of low-grade inflammation, and did not have a significant impact on SAT gene expression compared with MD and refined wheat products in participants with abdominal obesity.
Funding: This work was supported by a grant from Innovation Fund Denmark (MERITS 4105-00002B). Test powders were provided by Arla Foods Ingredients Group P/S, and wheat bran and cereal products were provided by Lantmännen Ek För. Enzymatic treatment of the wheat bran was performed by DuPont Nutrition Biosciences ApS.
Author contributions: All authors designed the study; ER and RFN conducted the research. ER performed the statistical analyses and had primary responsibility for writing the manuscript; all authors contributed to, and approved of, the final manuscript.
Acknowledgments: We thank Eva Mølgaard Jensen and Lene Trudsø for excellent technical and practical assistance with the study. We also thank Peter Reiter, Annemarie Kruse and Caroline Bruun Abild for their assistance with practical aspects.
Disclosures: The authors report no conflict of interests.
Appendix
Table
A1.
Gene symbols (with their commonly used aliases in parentheses), gene names, and the corresponding TaqMan Gene Expression Assay IDs |
|
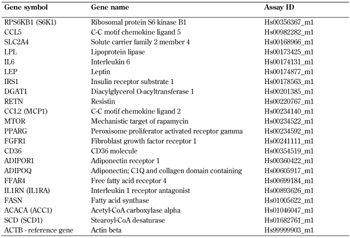 |
 |
References
- Wang X, Bao W, Liu J, OuYang YY, Wang D, Rong S, Xiao X, Shan ZL, Zhang Y, Yao P, Liu LG. Inflammatory markers and risk of type 2 diabetes. A systematic review and meta-analysis. Diabetes Care 2013. 36(1):166-175. [DOD] [CrossRef]
- Berg AH, Scherer PE. Adipose tissue, inflammation, and cardiovascular disease. Circ Res 2005. 96(9):939-949. [DOD] [CrossRef]
- Esser N, Legrand-Poels S, Piette J, Scheen AJ, Paquot N. Inflammation as a link between obesity, metabolic syndrome and type 2 diabetes. Diabetes Res Clin Pract 2014. 105(2):141-150. [DOD] [CrossRef]
- Ritchie SA, Connell JM. The link between abdominal obesity, metabolic syndrome and cardiovascular disease. Nutr Metab Cardiovasc Dis 2007. 17(4):319-326. [DOD] [CrossRef]
- Lemieux I, Pascot A, Prud'homme D, Almeras N, Bogaty P, Nadeau A, Bergeron J, Despres JP. Elevated C-reactive orotein. Another component of the atherothrombotic profile of abdominal obesity. Arterioscler Thromb Vasc Biol 2001. 21(6):961-967. [DOD] [CrossRef]
- Kim CS, Park HS, Kawada T, Kim JH, Lim D, Hubbard NE, Kwon BS, Erickson KL, Yu R. Circulating levels of MCP-1 and IL-8 are elevated in human obese subjects and associated with obesity-related parameters. Int J Obes 2006. 30:1347. [DOD] [CrossRef]
- Tsigos C, Kyrou I, Chala E, Tsapogas P, Stavridis JC, Raptis SA, Katsilambros N. Circulating tumor necrosis factor alpha concentrations are higher in abdominal versus peripheral obesity. Metabolism 1999. 48(10):1332-1335. [DOD] [CrossRef]
- Manning PJ, Sutherland WH, McGrath MM, De Jong SA, Walker RJ, Williams MJ. Postprandial cytokine concentrations and meal composition in obese and lean women. Obesity 2008. 16(9):2046-2052. [DOD] [CrossRef]
- Schwander F, Kopf-Bolanz KA, Egger L, Chollet M, Portmann R, Vergeres G, Buri C, Laederach K, Piya MK, McTernan PG, et al. A dose-response strategy reveals differences between normal-weight and obese men in their metabolic and inflammatory responses to a high-fat meal. J Nutr 2014. 144(10):1517-1523. [DOD] [CrossRef]
- Blackburn P, Despres JP, Lamarche B, Tremblay A, Bergeron J, Lemieux I, Couillard C. Postprandial variations of plasma inflammatory markers in abdominally obese men. Obesity 2006. 14(10):1747-1754. [DOD] [CrossRef]
- Yannakoulia M, Yiannakouris N, Melistas L, Kontogianni MD, Malagaris I, Mantzoros CS. A dietary pattern characterized by high consumption of whole-grain cereals and low-fat dairy products and low consumption of refined cereals is positively associated with plasma adiponectin levels in healthy women. Metabolism 2008. 57(6):824-830. [DOD] [CrossRef]
- Panagiotakos DB, Pitsavos CH, Zampelas AD, Chrysohoou CA, Stefanadis CI. Dairy products consumption Is associated with decreased levels of inflammatory markers related to cardiovascular disease in apparently healthy adults: The ATTICA Study. J Am Coll Nutr 2010. 29(4):357-364. [DOD] [CrossRef]
- Qi L, van Dam RM, Liu S, Franz M, Mantzoros C, Hu FB. Whole-grain, bran, and cereal fiber intakes and markers of systemic inflammation in diabetic women. Diabetes Care 2006. 29(2):207-211. [DOD] [CrossRef]
- Wannamethee SG, Whincup PH, Thomas M, Sattar N. Associations between dietary fiber and inflammation, hepatic function and risk of type 2 diabetes in older men: potential mechanisms for benefits of fiber on diabetes risk. Diabetes Care 2009. 32(10):1823-1825. [DOD] [CrossRef]
- Buyken AE, Goletzke J, Joslowski G, Felbick A, Cheng G, Herder C, Brand-Miller JC. Association between carbohydrate quality and inflammatory markers: systematic review of observational and interventional studies. Am J Clin Nutr 2014. 99(4):813-833. [DOD] [CrossRef]
- Zhou LM, Xu JY, Rao CP, Han S, Wan Z, Qin LQ. Effect of whey supplementation on circulating C-reactive protein: A meta-analysis of randomized controlled trials. Nutrients 2015. 7(2):1131-1143. [DOD] [CrossRef]
- Calder PC, Ahluwalia N, Brouns F, Buetler T, Clement K, Cunningham K, Esposito K, Jönsson LS, Kolb H, Lansink M, et al. Dietary factors and low-grade inflammation in relation to overweight and obesity. Br J Nutr 2011. 106(S3):S1-S78. [DOD] [CrossRef]
- Ouchi N, Parker JL, Lugus JJ, Walsh K. Adipokines in inflammation and metabolic disease. Nature Reviews. Immunology 2011. 11(2):85-97. [DOD]
- Markova M, Pivovarova O, Hornemann S, Sucher S, Frahnow T, Wegner K, Machann J, Petzke KJ, Hierholzer J, Lichtinghagen R. Isocaloric diets high in animal or plant protein reduce liver fat and inflammation in individuals with type 2 diabetes. Gastroenterology 2017. 152(3):571-585. [DOD] [CrossRef]
- Kallio P, Kolehmainen M, Laaksonen DE, Kekäläinen J, Salopuro T, Sivenius K, Pulkkinen L, Mykkänen HM, Niskanen L, Uusitupa M, Poutanen KS. Dietary carbohydrate modification induces alterations in gene expression in abdominal subcutaneous adipose tissue in persons with the metabolic syndrome: the FUNGENUT Study. Am J Clin Nutr 2007. 85(5):1417-1427. [DOD] [CrossRef]
- Rakvaag E, Fuglsang-Nielsen R, Bach Knudsen KE, Landberg R, Johannesson Hjelholt A, Sondergaard E, Hermansen K, Gregersen S. Whey protein combined with low dietary fiber improves lipid profile in subjects with abdominal obesity: a randomized, controlled trial. Nutrients 2019. 11(9):2091. [DOD] [CrossRef]
- Saarinen MT, Lahtinen SJ, Sorensen JF, Tiihonen K, Ouwehand AC, Rautonen N, Morgan A. Treatment of bran containing bread by baking enzymes; effect on the growth of probiotic bacteria on soluble dietary fiber extract in vitro. Biosci Biotechnol Biochem 2012. 76(6):1135-1139. [DOD] [CrossRef]
- Livak KJ, Schmittgen TD. Analysis of relative gene expression data using real-time quantitative PCR and the 2(-delta delta)CT method. Methods 2001. 25(4):402-408. [DOD] [CrossRef]
- Benjamini Y, Hochberg Y. Controlling the false discovery rate: a practical and powerful approach to multiple testing. J R Stat Soc Series B Stat Methodol 1995. 57(1):289-300. [DOD]
- Kopf JC, Suhr MJ, Clarke J, Eyun SI, Riethoven JJM, Ramer-Tait AE, Rose DJ. Role of whole grains versus fruits and vegetables in reducing subclinical inflammation and promoting gastrointestinal health in individuals affected by overweight and obesity: a randomized controlled trial. Nutr J 2018. 17:72. [DOD] [CrossRef]
- Vitaglione P, Mennella I, Ferracane R, Rivellese AA, Giacco R, Ercolini D, Gibbons SM, La Storia A, Gilbert JA, Jonnalagadda S, et al. Whole-grain wheat consumption reduces inflammation in a randomized controlled trial on overweight and obese subjects with unhealthy dietary and lifestyle behaviors: role of polyphenols bound to cereal dietary fiber. Am J Clin Nutr 2015. 101(2):251-261. [DOD] [CrossRef]
- Pedersen A, Christensen T, Matthiessen J, Knudsen V, Rosenlund-Sørensen M, Biltoft-Jensen A, Hinsch HJ, Ygil KH, Korup K, Saxholt E, et al. Dietary habits in Denmark 2011-2013. Main results. DTU Fodevareinstituttet. Soborg, Denmark, 2015. [DOD]
- Hoevenaars FPM, Esser D, Schutte S, Priebe MG, Vonk RJ, van den Brink WJ, van der Kamp JW, Stroeve JHM, Afman LA, Wopereis S. Whole grain wheat consumption affects postprandial inflammatory response in a randomized controlled trial in overweight and obese adults with mild hypercholesterolemia in the Graandioos Study. J Nutr 2019. In press. [DOD]
- Lutsey PL, Jacobs DR, Kori S, Mayer-Davis E, Shea S, Steffen LM, Szklo M, Tracy R. Whole grain intake and its cross-sectional association with obesity, insulin resistance, inflammation, diabetes and subclinical CVD: The MESA Study. Br J Nutr 2007. 98(2):397-405. [DOD] [CrossRef]
- Björck I, Östman E, Kristensen M, Mateo Anson N, Price RK, Haenen GR, Havenaar R, Bach Knudsen KE, Frid A, Mykkänen H, et al. Cereal grains for nutrition and health benefits: overview of results from in vitro, animal and human studies in the HEALTHGRAIN project. Trends Food Sci Technol 2012. 25(2):87-100. [DOD] [CrossRef]
- Knudsen KE, Hartvigsen ML, Hedemann MS, Hermansen K. Mechanisms whereby whole grain cereals modulate the prevention of type 2 diabetes. In: molecular nutrition and diabetes. Mauricio D (ed.). Elsevier 2016, pp. 87-103. [DOD]
- Mateo Anson N, Hemery YM, Bast A, Haenen GR. Optimizing the bioactive potential of wheat bran by processing. Food Funct 2012. 3(4):362-375. [DOD] [CrossRef]
- Mateo Anson N, Aura AM, Selinheimo E, Mattila I, Poutanen K, Berg R, Havenaar R, Bast A, Haenen GR. Bioprocessing of wheat bran in whole wheat bread increases the bioavailability of phenolic acids in men and exerts antiinflammatory effects ex vivo. J Nutr 2011. 141(1):137-143. [DOD] [CrossRef]
- Kang H, Lee MG, Lee JK, Choi YH, Choi YS. Enzymatically-processed wheat bran enhances macrophage activity and has in vivo anti-inflammatory effects in mice. Nutrients 2016. 8(4):188. [DOD] [CrossRef]
- de Aguilar-Nascimento JE, Silveira BR, Dock-Nascimento DB. Early enteral nutrition with whey protein or casein in elderly patients with acute ischemic stroke: a double-blind randomized trial. Nutrition 2011. 27(4):440-444. [DOD] [CrossRef]
- Pal S, Ellis V. The chronic effects of whey proteins on blood pressure, vascular function, and inflammatory markers in overweight individuals. Obesity 2010. 18(7):1354-1359. [DOD] [CrossRef]
- Bohl M, Bjornshave A, Gregersen S, Hermansen K. Whey and casein proteins and medium-chain saturated fatty acids from milk do not increase low-grade inflammation in abdominally obese adults. Rev Diabet Stud 2016. 13(2-3):148-157. [DOD] [CrossRef]
- Fekete AA, Giromini C, Chatzidiakou Y, Givens DI, Lovegrove JA. Whey protein lowers blood pressure and improves endothelial function and lipid biomarkers in adults with prehypertension and mild hypertension: results from the chronic Whey2Go randomized controlled trial. Am J Clin Nutr 2016. 104(6):1534-1544. [DOD] [CrossRef]
- Holmer-Jensen J, Karhu T, Mortensen LS, Pedersen SB, Herzig KH, Hermansen K. Differential effects of dietary protein sources on postprandial low-grade inflammation after a single high fat meal in obese non-diabetic subjects. Nutr J 2011. 10:115. [DOD] [CrossRef]
- Meier CA, Bobbioni E, Gabay C, Assimacopoulos-Jeannet Fo, Golay A, Dayer JM. IL-1 receptor antagonist serum levels are increased in human obesity: a possible link to the resistance to leptin? J Clin Endocrinol Metab 2002. 87(3):1184-1188. [DOD]
- Herder C, Brunner EJ, Rathmann W, Strassburger K, Tabak AG, Schloot NC, Witte DR. Elevated levels of the anti-inflammatory interleukin-1 receptor antagonist precede the onset of type 2 diabetes: The Whitehall II Study. Diabetes Care 2009. 32(3):421-423. [DOD] [CrossRef]
- Uusitupa M, Hermansen K, Savolainen MJ, Schwab U, Kolehmainen M, Brader L, Mortensen LS, Cloetens L, Johansson-Persson A, Onning G, et al. Effects of an isocaloric healthy Nordic diet on insulin sensitivity, lipid profile and inflammation markers in metabolic syndrome - a randomized study (SYSDIET). J Intern Med 2013. 274(1):52-66. [DOD] [CrossRef]
- Hay N, Sonenberg N. Upstream and downstream of mTOR. Genes Dev 2004. 18(16):1926-1945. [DOD] [CrossRef]
- Kimball SR, Jefferson LS. Regulation of global and specific mRNA translation by oral administration of branched-chain amino acids. Biochem Biophys Res Commun 2004. 313(2):423-427. [DOD] [CrossRef]
- Tremblay F, Krebs M, Dombrowski L, Brehm A, Bernroider E, Roth E, Nowotny P, Waldhäusl W, Marette A, Roden M. Overactivation of S6 kinase 1 as a cause of human insulin resistance during increased amino acid availability. Diabetes 2005. 54(9):2674-2684. [DOD] [CrossRef]
- Yoon MS. The emerging role of branched-chain amino acids in insulin resistance and metabolism. Nutrients 2016. 8(7):E405. [DOD] [CrossRef]
- Newgard CB, An J, Bain JR, Muehlbauer MJ, Stevens RD, Lien LF, Haqq AM, Shah SH, Arlotto M, Slentz CA. A branched-chain amino acid-related metabolic signature that differentiates obese and lean humans and contributes to insulin resistance. Cell Metab 2009. 9(4):311-326. [DOD] [CrossRef]
- Batch BC, Hyland K, Svetkey LP. Branch chain amino acids: biomarkers of health and disease. Curr Opin Clin Nutr Metab Care 2014. 17(1):86-89. [DOD]
- Sluijs I, Beulens JW, van der A DL, Spijkerman AM, Grobbee DE, van der Schouw YT. Dietary intake of total, animal, and vegetable protein and risk of type 2 diabetes in the European Prospective Investigation into Cancer and Nutrition (EPIC)-NL Study. Diabetes Care 2010. 33(1):43-48. [DOD] [CrossRef]
- Rietman A, Schwarz J, Tome D, Kok FJ, Mensink M. High dietary protein intake, reducing or eliciting insulin resistance? Eur J Clin Nutr 2014. 68:973-979. [DOD]
- Weickert MO, Roden M, Isken F, Hoffmann D, Nowotny P, Osterhoff M, Blaut M, Alpert C, Gögebakan Ö, Bumke-Vogt C, et al. Effects of supplemented isoenergetic diets differing in cereal fiber and protein content on insulin sensitivity in overweight humans. Am J Clin Nutr 2011. 94(2):459-471. [DOD] [CrossRef]
- Gran P, Larsen AE, Bonham M, Dordevic AL, Rupasinghe T, Silva C, Nahid A, Tull D, Sinclair AJ, Mitchell CJ. Muscle p70S6K phosphorylation in response to soy and dairy rich meals in middle aged men with metabolic syndrome: a randomised crossover trial. Nutr Metab (Lond) 2014. 11(1):46. [DOD] [CrossRef]
- Huang X, Yang Z. Resistin's, obesity and insulin resistance: the continuing disconnect between rodents and humans. J Endocrinol Invest 2016. 39(6):607-615. [DOD] [CrossRef]
- Vogel C, Marcotte EM. Insights into the regulation of protein abundance from proteomic and transcriptomic analyses. Nat Rev Genet 2012. 13:227. [DOD] [CrossRef]
- Vohl MC, Sladek R, Robitaille J, Gurd S, Marceau P, Richard D, Hudson TJ, Tchernof A. A survey of genes differentially expressed in subcutaneous and visceral adipose tissue in men. Obes Res 2004. 12(8):1217-1222. [DOD] [CrossRef]
|